Table of Contents
Diagnostics of SARS-CoV-2
Created by: Anastasiya Dudnyk, Gurkaran Chhaggar, Jessica Bensky, Lan Yang, Teng Yi Huang
SARS-CoV-2 Background Information
The novel coronavirus is an enveloped virus with a positive single stranded RNA genome. Its viral genome encodes many important proteins among which include RNA-dependent RNA polymerase (RdRp), spike (S), membrane (M), envelope (E), and the nucleocapsid (N) proteins (Malik, 2020). The viral entry into host cells is facilitated by the attachment of the viral S protein to the Angiotensin-converting enzyme 2 (ACE2) membrane receptor on host cells. Upon binding, the viral membrane fuses with the host cell membrane and the virus is endocytosed. It is believed that the virus was spread to humans through zoonotic transmission. The origin of the novel virus is unknown, but consistently bats were considered as the natural host for the novel coronavirus. It was postulated that the virus was transmitted from bats to several intermediate hosts before it was transmitted to humans (Jackson et al., 2022). On February 11, 2020, the novel coronavirus was identified as severe acute respiratory syndrome coronavirus 2 (SARS-CoV-2) by the World Health Organization (WHO). At the same time, the WHO referred to the disease caused by SARS-CoV-2 as the coronavirus disease of 2019, or COVID-19 (Lai et al., 2020).
Clinical symptoms of COVID-19 include fever, fatigue, dry cough, and decreased sense of smell and taste, and in severe cases, dyspnea. Based on patient imaging, especially anatomic analysis, COVID-19 can cause damage to multiple organs, including the lungs and liver. Most patients have a good prognosis, but some severe cases may develop acute respiratory distress syndrome or septic shock, and may lead to death. In some cases, COVID-19 positive individuals have no relevant clinical manifestations and no CT imaging evidence of COVID-19 during the course of disease; these individuals are termed asymptomatic infected persons (Zaim et al., 2020).
SARS-CoV-2 transmission occurs in a variety of ways. In addition to respiratory droplets and contact, the virus can also be transmitted through aerosols, the digestive tract, and from mother to child (Meyerowitz et al., 2020). Based on the current epidemiological investigation, the incubation period of the disease is 1 to 14 days, most of which are 3 to 7 days (Lauer et al., 2019). SARS-CoV-2 sheds during the incubation period where the hosts are pre-symptomatic or asymptomatic (Meyerowitz et al., 2020). The viral load in the upper respiratory tract samples peaks at the first week of symptom onset and declines gradually thereafter (Cevik et al., 2021). Due to its various channels of transmission and strong infectivity, therefore, controlling novel coronavirus transmission has been more difficult than other coronavirus transmissions, such as SARS. This has been one of the important reasons for the high global infection rate. Early detection, isolation, diagnosis, and treatment are the top priorities for this epidemic prevention and control (Meyerowitz et al., 2020).
Diagnostics
What are Diagnostics?
Diagnosis is the process of determining the nature of a disease and distinguishing it from other possible conditions. In terms of COVID-19, the confirmation of the diagnosis is done via tests. Current strategies for detecting COVID-19 include: testing for the virus’s nucleic acid, or testing for antibodies that the immune system produces. That is, etiological testing and serological testing, respectively. Multiple testing methods have been developed with various performance characteristics, such as providing quick results (rapid antigen testing) and highly sensitive results (RT-qPCR). Having a diagnostic test allows public health authorities to separate the infected from the uninfected so that public health authorities can take measures to ensure that infected people stay out of circulation to prevent further spread (Kevadiya et al., 2021).
Diagnostic Techniques
Enzyme-linked immunosorbent assay (ELISA)
Enzyme-linked immunosorbent assay also known as ELISA is a serology test for COVID-19. It tests the amount of a certain antibody present in the blood and is a good method to detect previous infection, especially with asymptomatic COVID-19 infections. Most ELISAs for COVID-19 test the presence of IgG and IgM antibodies which are the main responders to infection. How the ELISA test works is pictured in Figure 3. Firstly, either the SARS-Cov-2 spike protein or recombinant N-protein is adsorbed to a solid phase. The spike protein on the outside membrane of SARS-Cov-2 facilitates the attachment of the virus to host cells and RNA entry (Scudellari, 2021). Recombinant N-protein is a phosphoprotein that binds to viral RNA creating a nucleocapsid which helps with viral replication (Huang et al., 2004). Next the patient sample is added and any reactive antibodies will bind to spike protein or N-protein. All unbound proteins are washed off and an antibody specific to the COVID-19 antibody is added. This second antibody contains an enzyme that can be activated by a chemical which causes the enzyme to create a colorimetric signal. In this way, the amount of antibody can be measured in the sample.
Advantages/Limitations
ELISA is the main serology method used by the CDC and there are many production companies, the best being Bio-Rad Platelia SARS-CoV-2 Total Ab, which has a sensitivity of 98% and specificity of 99% (FDA, 2021). There was also a study performed in May 2021 that determined that the ELISA had the best test accuracy out of many serological diagnostic techniques tested (Vengesai et al., 2021). Some of the limitations of ELISA are its longer turn-around time and the need for a lab setting to perform the test which limits its availability to the public. Although it can test for previous infection, it cannot detect very early infection as the antibodies would not yet be at high concentrations in order to be detected.
Antigen Lateral Flow Assay (LFA)
An antigen lateral flow assay (LFA) is a test that can detect the presence of an antigen of interest in a complex mixture. The test is compatible with various samples, such as saliva or tissue from a nasopharyngeal or oropharyngeal swab (Zhou et al., 2021).
How it works: As shown in Figure 4, a sample is added onto an absorbent sample pad, which contains a buffer that prepares the sample so that it can interact with the detection system. The sample then travels through various zones of polymeric strips in the conjugate release pad, which have molecules that can interact with the analyte of interest. Specifically, these molecules can be antibodies specific to an antigen. They are conjugated to particles which allow for visual detection, such as nanozymes or fluorescent microspheres. Then the sample, with its targeted antigens bound to the conjugated antibody, travel into the detection zone. This is a porous membrane that has antibodies stationed in lines. A signal on the control line indicates that the sample flowed correctly through the strip. If only the control line has a signal, the antigen of interest is not present in the sample (a negative test result). Antibodies specific to the antigen of interest are on a test line. Therefore, if there is a signal on both lines, the antigen of interest is present in the sample (a positive test result). Finally, there is an absorbent pad at the end of the strip to soak up any excess liquid and prevent backflow (Koczula & Gallotta, 2016).
Antigen LFA for COVID-19 Detection: Antigen LFA can be used to detect SARS-CoV-2 protein in respiratory secretions, such as the S or N protein (Zhou et al., 2021).
- S protein: the surface of SARS-CoV-2 has several glycosylated S proteins on its surface, which bind to the angiotensin-converting enzyme II (ACE2) host cell receptor to mediate entry into the virus. An antibody sandwich immunoassay can be used to detect the S protein. For example, an anti-S protein antibody conjugated to a nanozyme label can be added to the conjugate release pad to bind S protein in the patient sample. Then in the detection zone, the test line has an ACE2 probe which binds the receptor binding domain (RBD) of the S-protein, forming a sandwich. There are also anti-IgG antibodies (bind to IgG) on the control line, which bind the conjugated anti-S protein antibody to confirm proper flow through the test. The nanozyme label allows for visual detection of coloured lines, which can be seen in Figure 5 (Zhou et al., 2021).
- N protein: the N protein is the main structural protein of SARS-CoV-2, which can be exposed or released during virus assembly. Therefore, it can be used for early detection of SARS-CoV-2 via antigen LFA using a double-antibody sandwich approach, similar to that for the S protein. For example, anti-SARS-CoV-2 N protein monoclonal antibody-1 can be immobilized on the test line, with anti-SARS-CoV-2 N protein monoclonal antibody-2 conjugated with fluorescent microspheres as a detection probe. The N protein antigen binds the labelled monoclonal antibody-2 in the conjugate release pad, followed by the monoclonal antibody-1 on the test line, forming a sandwich. There are also anti-IgG antibodies on the control line to confirm proper flow through the test. After 15 minutes, a light source and photoelectric sensor can be used to detect a fluorescent signal, as is shown in Figure 6 (Zhou et al., 2021).
Advantages/Limitations
A main advantage of antigen LFA as a diagnostic test is that it can produce rapid results in as little as 5-30 minutes. Additionally, compared to other methods such as RT-qPCR, it is relatively cheap to produce. Lastly, the antigen LFA test is portable and simple to perform, making it excellent for point-of-care testing (Koczula & Gallotta, 2016). A limitation of the antigen LFA for SARS-CoV-2 detection is that if the S or N protein concentration is too low, the test will show a false negative result (Zhou et al., 2021).
RT-qPCR
What is it?
Reverse transcriptase-quantitative polymerase chain reaction (RT-qPCR) is a nucleic acid detection based technique that combines reverse transcription (RT) with quantitative polymerase chain reaction (qPCR). It is characterized by its high sensitivity and specificity and is considered as the “gold standard” for viral detection. It is also the main diagnostic technique used for the detection of SARS-CoV-2 (Teymouri et al., 2021). RT-qPCR is categorized into 2 types: one step assay and two step assay. One step assay combines RT and qPCR in a single reaction while two step assay separates the RT and qPCR steps into separate reactions (Wacker & Godard, 2005). Both categories of RT-qPCR are widely used for SARS-CoV-2 diagnostics depending on the region and government agencies.
How does it work?
First, viral RNA is extracted from the collected patient samples through the addition of buffers, detergents and salts followed by the centrifugation of the mixture. During this step, cellular RNase is also inactivated by detergents and thermal treatments to prevent RNA denaturation. The purified viral RNAs are then reverse transcribed into complementary DNA (cDNA) by reverse transcriptase and the cDNA samples will serve as the template for the qPCR reaction. Before conducting qPCR, primers are designed to target the specific SARS-CoV-2 genes such as genes that encode RdRp, E and N proteins. The cDNA, RNA primer, Taq DNA polymerase (a thermostable DNA polymerase), deoxynucleotide triphosphates and qPCR buffers are all combined into a tube, the tube is then placed into a thermocycler and starts the process of qPCR. The optimal qPCR protocols will differ depending on different target genes and reagents. In general, within each cycle, the temperature oscillates between 70°C and 90°C and at the end of each cycle the number of RNA replicates is doubled. At 70°C, DNA polymerase synthesizes a new strand of DNA from the template which gives rise to a double-strand DNA. At 95 °C, these strands separate from each other to give twice the number of single strand templates for subsequent rounds of DNA replication. This cycle is repeated several times until the number of cDNA replicates reach an amount capable of being detected by spectrofluorimetric techniques. Based on the fluorescent signal change that correlates with the amount of cDNA, the spectrofluorimetric technique offers a readout of the cDNA amplification on a computer (Teymouri et al., 2021).
Advantages
- When performed correctly, RT-qPCR is highly accurate with a sensitivity and specificity greater than 95%. Research demonstrates that RT-qPCR is less likely to yield false negatives compared to other widespread testing modalities like rapid antigen tests (Tahamtan & Ardebili, 2020).
- RT-qPCR can test if an individual has an active COVID-19 infection, whereas other techniques can only test for the presence of antibodies. Non-infectious patients are still seropositive and will display a positive result when tested with other techniques (Zhang et al., 2021).
- RT-qPCR positive individuals were more likely to report symptoms than those who were RT-qPCR negative but seropositive. This has significant epidemiological relevance for controlling viral spread (Zhang et al., 2021).
Limitations
- The genetic diversity and rapid evolution of the SARS-CoV-2 viral genome gives rise to mutations in the probe target region that cause mismatches between the RT-qPCR primers and probe target sequences which leads to a decrease in assay performance and potential false-negative results (Phan, 2020).
- The false-negative rate of RT-qPCR is significant and varies across different specimens and time periods. Different sampling sites from each patient present different viral loads, false negative results are inevitable and suboptimal sample sites may result in greater false negatives. Yang et al., reported a false negative rate of 11 % for seputum, 27 % for nasal, and 40 % for throat swabs within the first seven days from onset of illness in 213 COVID-19 positive patients. The false-negative rate of the test also changes over time depending on when the samples were obtained from the onset of symptoms (Yang et al., 2020). In a study of 1330 confirmed cases, the median false-negative rate reduced gradually from 100 % to 20 % during days 3 and 4 post-onset of symptoms and returned to 66% on day 21 post-onset of symptoms (Kucirka et al., 2020).
- The RT-qPCR procedure for SARS-CoV-2-infected samples consists of several steps, and requires expensive laboratory equipment that makes the process tedious and difficult to be conducted outside of a laboratory setting. The procedure also requires trained professionals. All these factors contribute to the relatively high cost and long turnaround time of RT-qPCR (Teymouri et al., 2021).
- It has been reported that RT-qPCR continues to display positive results in patients who have recovered from COVID-19 (false positive results). In one case report study SARS-CoV-2 RT-qPCR continued to detect the virus until the 63rd day from the onset of symptoms. It is suggested that the reason that RT-qPCR could give a positive result is by detecting viral RNA remnants in serum samples long after the infectious virus had disappeared. Therefore, it is possible that the RT-qPCR result was positive even after the infectious virus had been neutralized by the immune system (Teymouri et al., 2021).
Electrochemical aptamer-based sensors
Aptamers
Aptamers are short pieces of single stranded DNA that are able to bind to targets. DNA is used for aptamers because it is less prone to degradation making it more stable than RNA. The single stranded DNA harbours complementarity within itself, allowing it to fold and form a tertiary structure (Figure 1). Aptamers are able to bind to their targets using this tertiary structure and are able to do so with high affinity and specificity through non-covalent interactions as shown in the figure below (Xing et al., 2014).
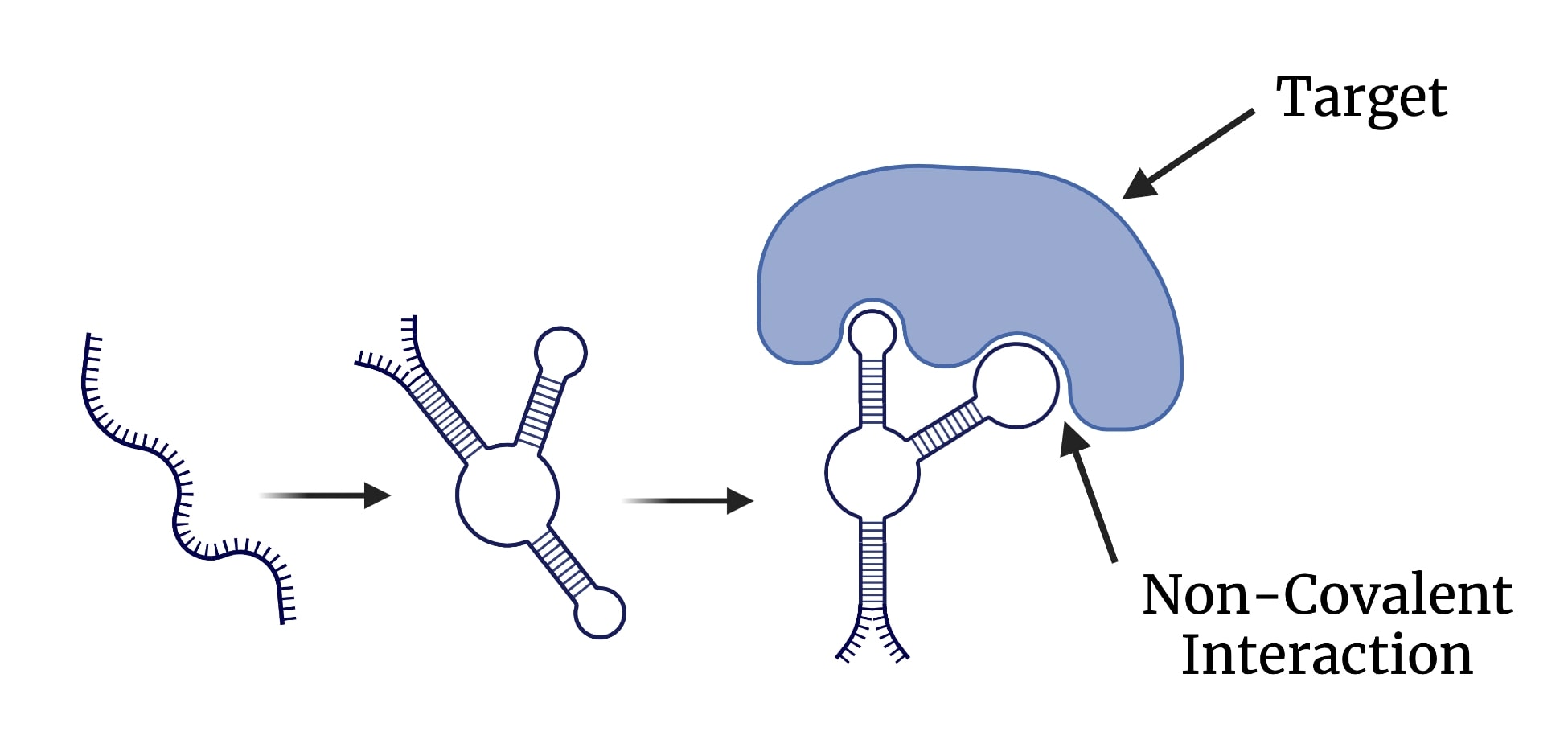
Figure 8: Illustration of DNA aptamers and their binding characteristics. Image created using BioRender.
Aptamers are selected for using a method called SELEX (Systematic Evolution of Ligands by Exponential Enrichment) (Tuerk & Gold, 1990). First, there is a random library of DNA aptamers that can be purified to yield a single type of aptamer that binds to a specific ligand. The library of aptamers is added to a column that harbours the target ligand (e.g., the SARS-CoV-2 spike protein) in a buffer solution. The aptamers that have affinity to the ligand stay in the column while the ones that do not, are washed away. Then an elution buffer is passed through the column, which breaks the interactions between the ligands and aptamers. The resulting aptamer containing eluent is amplified using PCR (polymerase chain reaction - gives more copies of the aptamers). The previous steps can be repeated multiple times to enrich the pool of aptamers (Zhuo et al, 2017). The figure below depicts the steps described above.
Electrochemical aptamer-based sensors
An electrochemical aptamer-based sensor is a relatively new diagnostic technique that can be used in the detection of SARS-CoV-2 (Idili et al., 2021). This technology is not restricted to SARS-CoV-2, but can also be used in the detection of many other pathogens. Further, this diagnostic technique is able to provide results in a single step and can be used as a quantitative method with response times ranging between a few seconds to 5 minutes (Idili et al., 2021). This system can be used with undiluted biological fluids, like saliva and blood (without buffers), which allows for efficient point-of-care testing of SARS-CoV-2 (Idili et al., 2021). DNA aptamers that are specific to the receptor binding domain (RBD) of the SARS-CoV-2 S protein are covalently attached to a gold electrode. The aptamers have a redox reporter that can generate a measurable electrochemical signal (Idili et al., 2021). Upon binding of the S protein, the aptamer changes it's conformation. The position of the redox reporter that is bound to the end of the aptamer is changed relative to the gold electrode. This in turn causes a change in the rate of electron transport. The system relies on the rate of electron transfer and when the redox reporter alters the rate of this transfer, there is a change in current, allowing for a detectable signal. This change in current signals that there is SARS-CoV-2 present in the sample. Figure 10 illustrates the conformational changes that occur to the aptamers upon binding of SARS-CoV-2 S protein and the change in position of the redox reporter.
Advantages/Limitations
Like many systems this technique does come with its advantages and limitations. Electrochemical aptamer-based sensors have a great range of advantages when compared to the previously discussed techniques. Perhaps one of the most important advantages of this technique is its ability to provide quick responses which are done in a single step. Further, the technique is easy to use since there are no reagents; biological fluids like saliva can be used; there is no dilution required; and detection at biological condition is achieved at a picomolar range (Idili et al., 2021). Another important advantage is that this technique can differentiate between different types of coronavirus RBDs and other proteins that are in the sample, as they will provide different changes in current. Lastly, this technique can be used with phones or portable electrochemical setups, which allows for optimal point-of-care testing. However, the one main drawback of this system is that it does not have a similar level of sensitivity when compared to RT-qPCR testing (Idili et al., 2021).
Conclusion
There exist many diagnostic techniques that can allow for the detection of not only SARS-CoV-2, but also a wide variety of other pathogens. There is a great overlap when diagnosing pathogens, but SARS-CoV-2 has given scientists the opportunity to explore novel diagnostic methods. Diagnosing SARS-CoV-2 in patients is crucial to stop the spread of this virus and end the pandemic. The techniques described above give insight into the current and potential diagnostic methods that can be used for detecting SARS-CoV-2. Each comes with its own advantages and limitations and their use depends on the situation and environment that it is being used in. Overall, as research and technology advances, there are going to be new diagnostic tools that can overcome the limitations of the ones listed above, allowing for more efficient detection of SARS-CoV-2 and a variety of other future pathogens.
References
Cascella, M., Rajnik, M., Aleem, A., et al. Features, Evaluation, and Treatment of Coronavirus (COVID-19) [Updated 2022 Jan 5]. In: StatPearls [Internet]. Treasure Island (FL): StatPearls Publishing; 2022 Jan-. [Figure, SARS- CoV 2 Structure. Contributed by Rohan Bir Singh, MD; Made with Biorender.com] Available from: https://www.ncbi.nlm.nih.gov/books/NBK554776/figure/article-52171.image.f3/
Cevik, M.,Tate, M., Lloyd, Ollie., Maraolo, A. E., Schafers, J., Ho, A. (2021). SARS-CoV-2, SARS-CoV, and MERS-CoV viral load dynamics, duration of viral shedding, and infectiousness: a systematic review and meta-analysis. The Lancet Microbe, 2(1), E13-22. https://doi.org/10.1016/S2666-5247(20)30172-5
Idili, A., Parolo, C., Alvarez-Diduk, R., & Merkoçi, A. (2021). Rapid and efficient detection of the SARS-CoV-2 spike protein using an electrochemical aptamer-based sensor. ACS sensors, 6(8), 3093-3101.
Jackson, C. B., Farzan, M., Chen, B. et al. (2022). Mechanisms of SARS-CoV-2 entry into cells. Nat Rev Mol Cell Biol, 23, 3–20. https://doi.org/10.1038/s41580-021-00418-x
Kevadiya, B. D., Machhi, J., Herskovitz, J. et al. (2021) Diagnostics for SARS-CoV-2 infections. Nat Mater, 20, 593-605. https://doi.org/10.1038/s41563-020-00906-z
Koczula, K. M., & Gallotta, A. (2016). Lateral flow assays. Essays in Biochemistry, 60(1), 111–120. https://doi.org/10.1042/ebc20150012
Kucirka, L. M., Lauer, S. A., Laeyendecker, O., Boon, D., & Lessler, J. (2020). Variation in false-negative rate of reverse transcriptase polymerase chain reaction–based SARS-COV-2 tests by time since exposure. Annals of Internal Medicine, 173(4), 262–267. https://doi.org/10.7326/m20-1495
Lai, C., et al. (2020). Severe acute respiratory syndrome coronavirus 2 (SARS-CoV-2) and coronavirus disease-2019 (COVID-19): The epidemic and the challenges. Int J Antimicrob Agents, 55(3), 105924. doi:10.1016/j.ijantimicag.2020.105924
Lauer, S. A., Grant, K. H., Bi, Q., et al. (2020). The Incubation Period of Coronavirus Disease 2019 (COVID-19) From Publicly Reported Confirmed Cases: Estimation and Application. Ann Intern Med, 172, 577-582. doi:10.7326/M20-0504
Malik, Y. A. (2020). Properties of Coronavirus and SARS-CoV-2. Malays J Pathol, 42(1), 3-11. Retrieved from: https://pubmed.ncbi.nlm.nih.gov/32342926/
Meyerowitz, E. A., Richterman, A., Gandhi, R. T., et al. (2020). Transmission of SARS-CoV-2: A Review of Viral, Host, and Environmental Factors. Ann Intern Med, 174, 69-79. doi:10.7326/M20-5008
Phan, T. (2020). Genetic diversity and evolution of SARS-COV-2. Infection, Genetics and Evolution, 81, 104260. https://doi.org/10.1016/j.meegid.2020.104260
Tahamtan, A., & Ardebili, A. (2020). Real-time RT-PCR in COVID-19 detection: Issues affecting the results. Expert Review of Molecular Diagnostics, 20(5), 453–454. https://doi.org/10.1080/14737159.2020.1757437
Teymouri, M., Mollazadeh, S., Mortazavi, H., Naderi Ghale-noie, Z., Keyvani, V., Aghababaei, F., Hamblin, M. R., Abbaszadeh-Goudarzi, G., Pourghadamyari, H., Hashemian, S. M., & Mirzaei, H. (2021). Recent advances and challenges of RT-PCR tests for the diagnosis of COVID-19. Pathology - Research and Practice, 221, 153443. https://doi.org/10.1016/j.prp.2021.153443
Tuerk, C., & Gold, L. (1990). Systematic evolution of ligands by exponential enrichment: RNA ligands to bacteriophage T4 DNA polymerase. science, 249(4968), 505-510.
Wacker, M. J., & Godard, M. P. (2005). Analysis of one-step and two-step real-time RT-PCR using SuperScript III. Journal of biomolecular techniques : JBT, 16(3), 266–271.
Xing, H., Hwang, K., Li, J., Torabi, S. F., & Lu, Y. (2014). DNA aptamer technology for personalized medicine. Current opinion in chemical engineering, 4, 79-87
Yang, Y., Yang, M., Shen, C., Wang, F., Yuan, J., Li, J., Zhang, M., Wang, Z., Xing, L., Wei, J., Peng, L., Wong, G., Zheng, H., Wu, W., Liao, M., Feng, K., Li, J., Yang, Q., Zhao, J., … Liu, Y. (2020). Evaluating the accuracy of different respiratory specimens in the laboratory diagnosis and monitoring the viral shedding of 2019-ncov infections. https://doi.org/10.1101/2020.02.11.20021493
Zaim, S., Chong, J. H., Sankaranarayanan, V., & Harky, A. (2020). COVID-19 and Multiorgan Response. Curr Probl Cardiol, 45(8), 100618. https://doi.org/10.1016/j.cpcardiol.2020.100618
Zhang, Z., Bi, Q., Fang, S., Wei, L., Wang, X., He, J., Wu, Y., Liu, X., Gao, W., Zhang, R., Gong, W., Su, Q., Azman, A. S., Lessler, J., & Zou, X. (2021). Insight into the practical performance of RT-PCR testing for SARS-COV-2 using serological data: A cohort study. The Lancet Microbe, 2(2). https://doi.org/10.1016/s2666-5247(20)30200-7
Zhou, Y., Wu, Y., Ding, L., Huang, X., & Xiong, Y. (2021). Point-of-care covid-19 diagnostics powered by lateral flow assay. Trends in Analytical Chemistry, 145, 116452. https://doi.org/10.1016/j.trac.2021.116452
Zhuo, Z., Yu, Y., Wang, M., Li, J., Zhang, Z., Liu, J., … Zhang, B. (2017, October). Recent Advances in SELEX Technology and Aptamer Applications in Biomedicine. Retrieved from https://www.ncbi.nlm.nih.gov/pmc/articles/PMC5666824/.