Table of Contents
By: Sarah Choe, Tianjia Cui, Elias Espinosa, Rachel Guan, Tim Wang
SARS-CoV-2 Background
The disease COVID-19 is caused by the virus SARS-CoV-2 and has infected millions of individuals worldwide. It has killed around 5,700,000 people and has shown no signs of slowing down (“WHO Coronavirus (COVID-19) Dashboard”, 2022). COVID-19’s incredibly high infection and death rate support its characterization as one of the most infectious diseases in recent history. Originating in Wuhan China, the world health organization deemed the outbreak a Public Health Emergency of International Concern on 30 January 2020 (“WHO Timeline - COVID-19.”, 2020). It was later declared a pandemic on 11 March 2020 (“WHO Timeline - COVID-19.”, 2020). SARS-CoV-2 is an acronym for, Severe Acute Respiratory Syndrome CoronaVirus 2 (“Naming the coronavirus disease (COVID-19) and the virus that causes it.”, n.d.). Fittingly, this virus causes respiratory problems, shortness of breath, fever/high temperatures, and loss of taste and/or smell (Centers for Disease Control and Prevention, 2021). Additionally, in extreme cases, organ failure and myocarditis (Centers for Disease Control and Prevention, 2021). The severity of symptoms is variable between individuals, about 40% of infected people will be asymptomatic altogether (Ma et al., 2021). COVID-19 is most commonly transmitted through contaminated droplets and aerosols entering the mouth, nose, and rarely, the eyes (“Coronavirus disease (COVID-19): How is it transmitted?”, 2021).
SARS-CoV-2 Structure
The SARS-CoV-2 virus has 4 main structural protein components: the (S) spike protein, the (N) nucleocapsid protein, the (E) envelope protein, and the (M) membrane protein (Wang et al., 2020). The Spike transmembrane proteins protrude from the virion and help bind to the host receptor or receptors for attachment (Wang et al., 2020). It is incredibly important for the successful entry of the coronavirus into the host cell and thus, is an ideal target when designing therapeutic drugs. Adequate binding of the spike protein determines infectivity, host range, and pathogenesis of the virus (Wang et al., 2020).
The nucleocapsid protein is a structural protein that may bind to genomic RNA, is vital for virus assembly and transcription (Wang et al., 2020). Its main function is to package viral genomes into long, flexible, ribonucleoprotein complexes, a critical step for effective transmission (Wang et al., 2020). Studies show that mutations along the N protein can increase the infectivity of the SARS-CoV-2 virus by 50-fold (Syed et al., 2021).
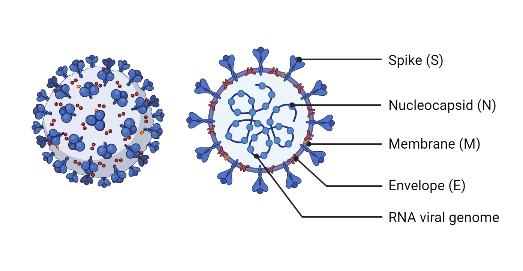
Figure 1: The structure of a SARS-CoV-2 virion. The (E) Envelope, (M) membrane, (N) nucleocapsid, (S) spike proteins are visualized. (The Economist, 2022).
The integral envelope protein plays many parts in the virus' life. It functions to assembly, budding, envelope formation, and pathogenesis (Schoeman & Fielding, 2019). The M protein is a transmembrane glycoprotein that can bind with other structural proteins to help them complete their function. Binding to N proteins aid in their viral assembly functionality by stabilizing the protein-RNA complex (Thomas, 2020).
SARS-CoV-2 Variants
Many SARS-CoV-2 variants have been found and explored over the past couple of years. Through multiple rounds of replication, beneficial mutations have occurred which changed different aspects of the virus (Bollinger & Ray, 2022). Most of the beneficial naturally selected mutations occur on the four structural proteins (M, E, N, S). Whereby the mutation increases the protein's affinity/efficiency at performing their tasks (Bollinger & Ray, 2022). Two of the variants, Delta and Omicron are known to be more contagious and infectious than the standard Alpha strain. This difference is due to specific amino acid substitutions on the spike protein subunit, giving the variant a higher affinity to host cell binding and thereby increasing the infection rate (Bollinger & Ray, 2022).
SARS-CoV-2 Vaccine
The main method in defending against COVID-19 is the utilization of vaccines. A vaccine is a biochemical tool used to treat infectious diseases. Currently, there are 4 main types of vaccines used to prevent the spread of COVID-19.
The Four Main Types of Covid-19 Vaccines
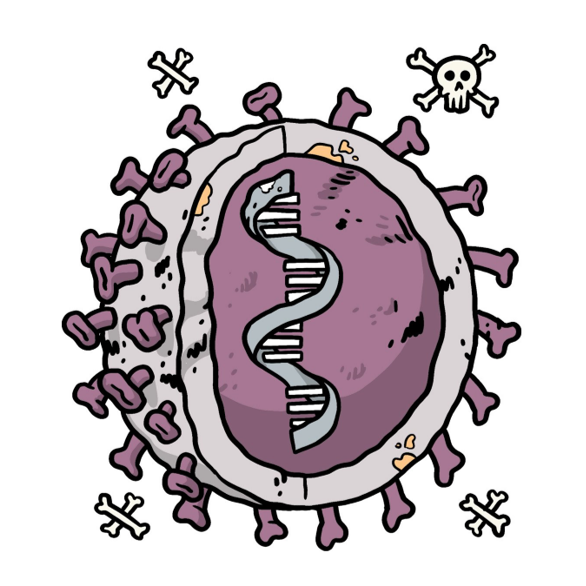
Figure 2: Representation of the content belonging to an inactivated vaccine. The genetic material coloured in grey represents genetic material that is inactive. Image adapted from (WHO, 2021).
Diverse SARS-CoV-2 vaccines can be divided into 4 main types: whole virus vaccines (inactivated and live attenuated), protein subunit vaccines, virus vector vaccines, and nucleic acid vaccines. The ultimate goal of these vaccines is to help people build immunity against the SARS-CoV-2 via antigen presentation. Some of them aim to bring the antigen into the body such as whole virus and protein subunit vaccines, while others rely on the body’s cells to make viral antigens such as viral vector and nucleic acid vaccines. The spike protein found on the surface of the virus is a popular target of interest as it helps the virus penetrate human cells.
Whole Virus Vaccine
The more conventional whole virus vaccine uses either a weakened or deactivated form of the virus to trigger an immune response. It comes in two main forms: inactivated vaccine and live-attenuated vaccine (Gavi, 2020).
Inactivated vaccines:
Inactivated vaccines contain non-infectious viruses whose genetic material has been destroyed via physical or chemical approaches like heat, chemicals, or radiation (Dong et al., 2020). The inactive viruses cannot infect cells and replicate, but can still trigger an immune response (Gavi, 2020).
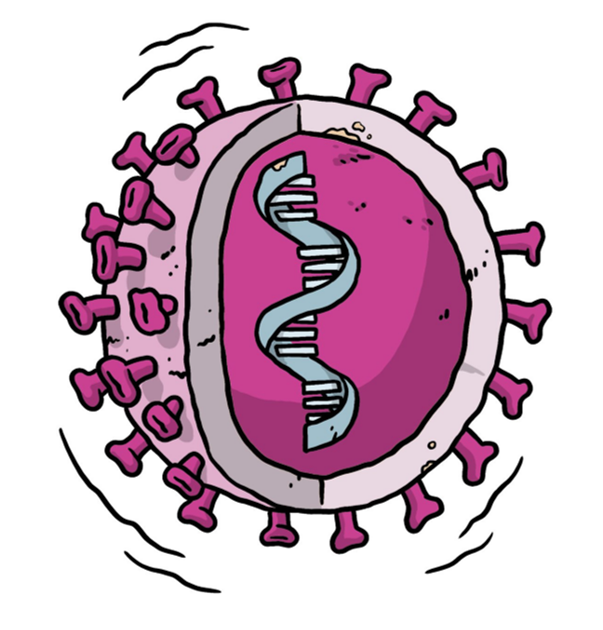
Figure 3: Representation of a live attenuated vaccine. The black line surrounding the virus represents its weakened state. Image adapted from (WHO, 2021).
The advantages of inactivated vaccines include presenting multiple viral proteins at once for immune recognition, stable expression of conformation-dependent antigenic epitopes, and easy production in large quantities owing to its well-established technology (Gavi, 2020; Dong et al., 2020). Examples of inactivated vaccine candidates include CoronaVac which is manufactured by Sinovac Biotech in China (Krammer, 2020).
Live attenuated vaccines:
Live attenuated vaccines use a genetically weakened version of the virus with limited capacity to grow and replicate but cannot cause illness disease and induce immune responses to a similar level of natural infection. (Krammer, 2020) Attenuation can be achieved by adapting the virus into unfavourable conditions via altering its culture environments (e.g. culturing them at lower temperature or in non-human cells) or genetically de-optimize viral codon (e.g. deleting genes that are responsible for counteracting innate immune recognition) (Krammer, 2020).
One importance merit of these vaccines is that they can be administrated intranasally to induce mucosal immune responses, which allows a direct protection to the upper respiratory tract—the major entry portal of the SARS-CoV-2 (Krammer, 2020). However, concerns have been raised that such vaccines may revert to virulence in some cases (Dong et al., 2020).
Protein Subunit and Virus-Like Particle Vaccines
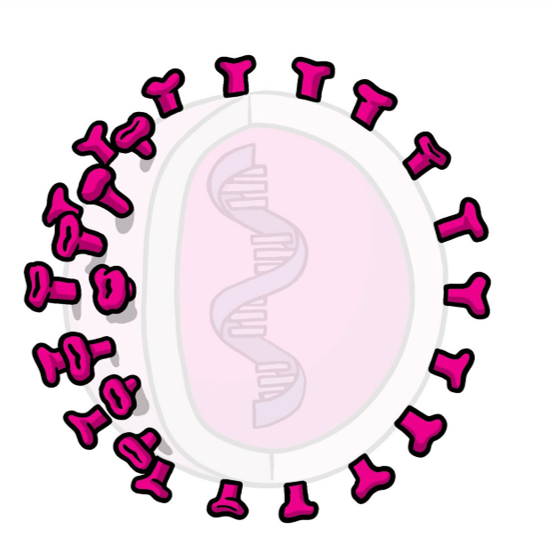
Figure 4: Representation of a protein subunit vaccine. The pink opaque spikes represented in the image displays a protein subunit that can stimulate an immune response. Image adapted from (WHO, 2021).
A protein subunit vaccine uses purified viral proteins that are specially selected for their ability to stimulate immune cells after injecting them into the host (Gavi, 2020). Since these viral subunits are incapable of triggering the disease, subunit vaccines are suitable for people with compromised immune systems (Gavi, 2020).
Nonetheless, since the selected viral components cannot display the full antigenic complexity of the virus or infect cells, subunit vaccines may only trigger antibody-mediated immune responses (Dong et al., 2020). This could limit its protective efficacy and cause unbalanced immune responses (Dong et al., 2020). Therefore, subunit vaccines could be used in combination with protein adjuvants or booster doses may be required.
Virus-like particles (VLPs) is another protein-based vaccine using proteins from the viral capsid (Dong et al., 2020) The VLPs vaccines of SARS-CoV-2 carry no genome but display the spike protein on their surface (Krammer, 2020). Owing to the repetitive structures which elicit robust humoral and cellular immune responses and the lack of genetic material, the VLPs vaccine is believed to be a safe and highly immunogenic vaccine platform (Prates-Syed et al., 2021).
Viral Vector Vaccine
Viral vector-based vaccines use a safe vector-like modified adenoviruses to deliver genetic code for antigens into human cells, which triggers an immune response without causing disease. In the case of COVID-19, the gene of interest was the spike proteins found on the surface of the coronaviruses (Gavi, 2020; Dong et al., 2020).
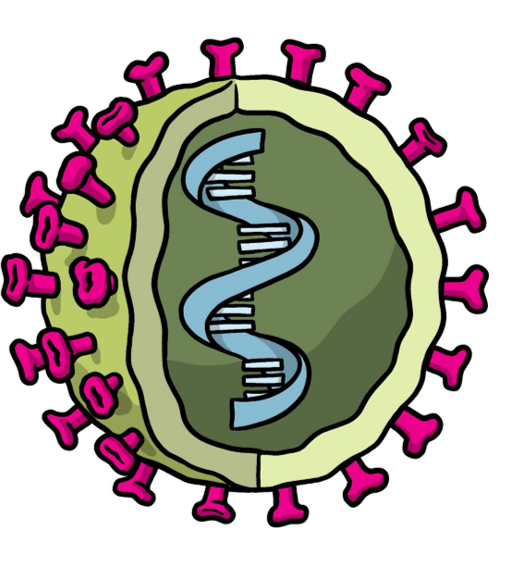
Figure 5: Representation of a viral vector vaccine. The green shell represents the vector (e.g. adenovirus) and the blue DNA represents the antigen gene. Image adapted from (WHO, 2021).
These vaccines differ from other conventional vaccines like whole virus or protein subunit vaccines as viral vector vaccines do not contain antigens, but rather infect and instruct the host cells to make large amounts of antigen for immune recognition. (Gavi, 2020; Dong et al., 2020)
One important advantage of such vaccines are that they trigger a strong cellular immune response from both T cells and antibody-producing B cells (Gavi, 2020). Nonetheless, “anti-vector immunity” that refers to people who previously experienced the virus vector and thus have immune responses against it, which could reduce the effectiveness of this vaccine approach (Gavi, 2020).
Furthermore, inactivated virus vector is another technology advancement in SARS-CoV-2 vaccine development where the virus vectors carry copies of the spike protein on their surface but have been chemically inactivated before use (Krammer, 2020). This inactivation process renders the vectors safer because it prevents viral replication even in an immunocompromised host and the amount of antigen presented can be standardized (Krammer, 2020).
Nucleic Acid (RNA and DNA) Vaccines
Nucleic acid vaccines deliver the genetic information of an antigen rather than the antigen itself. Depending on the vaccine, the genetic material could be DNA or RNA. In both cases, the nucleic acids will be transcribed into viral proteins by the protein-making machinery of the host cell, which then triggers an immune response. (Gavi, 2020; Dong et al., 2020) Similar to other vaccines, the spike protein has been the most common candidate for nucleic acid vaccine development.
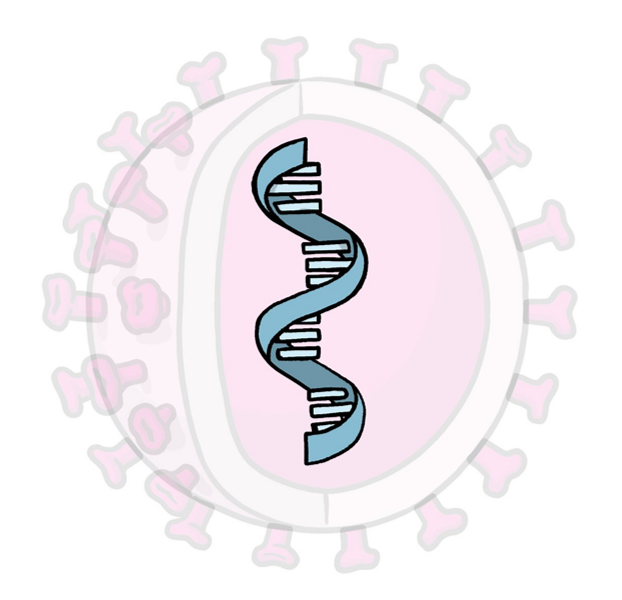
Figure 6: Representation of the content belonging to a nucleic acid vaccine. The blue genetic material represents mRNA or DNA. Image adapted from (WHO, 2021).
DNA vaccines use DNA plasmids to carry the antigen and are usually injected into the muscle. Although their great therapeutic potential resides in their ability to induce T-cell response and antibody production, one major disadvantage is that the DNA molecules must cross both the cellular and the nuclear membranes to be transcribed (Shukla, 2021).
In contrast, the mRNA vaccine only needs to cross the cell membrane to synthesize the viral proteins (Shukla, 2021). Therefore, the fact that DNA vaccines require an extra step of entering the cell nucleus to function confers generally low-level immunogenicity compared to mRNA vaccines.
Finally, RNA vaccines are a relatively recent development and thus, we do not have much clinical data about their long-term safety, effects, large-scale production, and long-term storage stability (Krammer, 2020). The RNA can be injected by itself, encapsulated within nanoparticles such as Pfizer’s mRNA-based vaccine. Another advantage of mRNA vaccine technology is that the vaccine can be produced completely in vitro yet ultra-cold storage is required (Krammer, 2020)
Development of mRNA Vaccines
Two of the three licensed vaccines in Canada are mRNA vaccines. These mrna-based vaccines are a result of advancements in RNA biology, immunology, lipid-based delivery systems, genetic engineering, chemical modification, and nanoparticle technology that allowed an accelerated development of these vaccines within a year of the pandemic (Granados-Riveron & Aquino-Jarquin, 2021).
Currently, the only approved model of mRNA vaccines for SARS-CoV-2 is the non-replicating mRNA model that only encodes the antigen. The structure of the non-replicating mrna is similar to that of mature eukaryotic mRNA which comprises of the antigen encoding open reading frame flanked by 5’ and 3’ untranslated regions, a 7-methyl guanosine 5’ cap, and a 3’ poly(A) tail. These aspects of mRNA have been optimized and adapted to the current SARS-CoV-2 vaccines to improve mRNA half-life, immunogenicity, stability, and the duration of protein expression (Granados-Riveron & Aquino-Jarquin, 2021). Additionally, the magnitude of protein expression can be increased by optimizing translation of the mRNA by utilizing synonymous codons that are recognized by multiple tRNA’s. Most notably, the mRNA-1273 and BNT162b2 vaccines contain a nucleoside modification where the in-vitro transcribed transcripts have a substitution of uridines for N1-methyl pseudouridine. This serves to increase translation efficiency while reducing the immunogenicity and degradability. This is the reason why the two mRNA vaccines are also referred to as nucleoside-modified SARS-CoV-2 mRNA-LNP vaccines (Granados-Riveron & Aquino-Jarquin, 2021).
Pfizer/BioNTech/Fosun Pharma (mRNA-BNT162b2/Comirnaty) COVID‑19 vaccine
BNT162b2 is one of the two candidates of the mRNA platform, developed in collaboration with Pfizer and Biopharmaceutical New Technologies (BioNTech) and Fosun Pharma. This vaccine contains a nucleoside-modified mRNA molecule that encodes the stabilized prefusion conformation of the SARS-CoV-2 Spike protein encapsulated in a LNP (Granados-Riveron & Aquino-Jarquin, 2021).
The first stage of its production involves cloning the SARS-CoV-2 segment spike protein gene into DNA plasmid vectors which are then propagated in E.coli. After four days of growth, the bacteria cultures are lysed, and the DNA plasmid is purified for around 10 days. The second stage involves the utilization of the DNA as a template for building the required mRNA strands (Granados-Riveron & Aquino-Jarquin, 2021). The plasmid DNA template required to produce in-vitro transcripts contains at least a bacteriophage promoter, an open-reading frame, a poly(A) sequence, and a unique restriction site for the plasmid's linearization to ensure defined termination of transcription. The transcripts are purified by liquid chromatography to separate the desired transcripts from the longer and shorter mRNA. Roughly 5-10 million doses of mRNA are transported to the next stage where the lipid nanoparticles are produced (Granados-Riveron & Aquino-Jarquin, 2021).
The mRNA sequence encodes the RBD of the spike protein, modified by a foldon trimerization domain to increase immunogenicity by the multivalent display. The sequence is 4284 nucleotides long with a molecular weight of 1388 kDA. It possesses a modified 5’ CAP1 structure, a 5’ UTR region derived from the highly expressed human gene alpha-globin, and an optimized downstream Kozack consensus GCCACC AUG instead of ACCAUG (where AUG is the start codon) (Granados-Riveron & Aquino-Jarquin, 2021). The ORF encodes the full-length spike protein of SARS-CoV-2(nucleotides 55-3879) and its signal peptide (55-102). The ORF is codon optimized with two proline substitutions at K986 and V987 which causes the spike protein to be in the prefusion-stabilized conformation to reduce its membrane fusion ability. This results in its increased expression and stimulation of neutralizing antibodies (Granados-Riveron & Aquino-Jarquin, 2021). It’s 3’ UTR possesses a combination of the Amino-terminal enhancer of split gene sequence and mtRNR1 mitochondrial 12S ribosomal RNA to increase protein expression and mRNA stability. The 3’ end is then completed with a 30-mer poly(A) tail, a 10-nucleotide linker sequence, and 70 other adenosine residues for further and prolonged protein expression (Granados-Riveron & Aquino-Jarquin, 2021). The modified nucleosides were incorporated by substituting the uridine residues for 1-methyl-3-pseudouridine prevent the activation of TLR7, TLR8, and other innate immune sensors that trigger IFN-I signaling to reduce pro-inflammatory response upon vaccination. This completely reduces the immunogenicity of the mRNA transcript by pattern recognition receptors and ribonucleases when released into the cytosol (Granados-Riveron & Aquino-Jarquin, 2021).
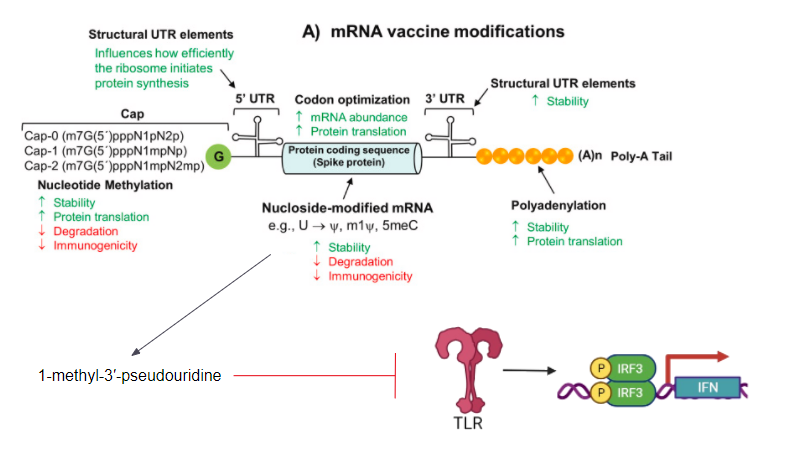
Figure 7: A list of all possible modifications and optimizations that can be made to the mRNA transcript (Granados-Riveron & Aquino-Jarquin, 2021).
Lipid Nanoparticles as a Delivery System for mRNA Vaccines
In order to achieve cellular uptake of mRNA from mRNA vaccines in-vivo, the mRNA requires safe, effective, and stable delivery systems that protect the nucleic acid from degradation by ribonucleases and other enzymes while facilitating mRNA release within the cell cytosol.
Liquid nanoparticles compose of an aqueous core surrounded by a lipid bilayer shell made from the combination of multiple lipids with distinct functions (Reichmuth et al., 2016). Early LNP formulations utilized cationic lipids to complex with the negatively charged RNA in the core however, permanent cationic lipids in circulation of the body were determined to be toxic. As a result, a new generation of ionizable lipids were utilized which contained amine groups. These would maintain a mild cationic surface charge at physiological pH to reduce nonspecific lipid-protein interactions while still facilitating mrna release in the cytosol of target cells (Reichmuth et al., 2016). The ionizable cationic lipids would then have their amine groups ionized upon being taken up by the acidic environment of the cell’s endosome. This would induce hexagonal phase structures between the lipid membranes and disrupt the late endosomal membrane. Finally, endosomal escape and cellular uptake of the mRNA into the cytoplasm would be achieved (Reichmuth et al., 2016). Although the ionizable cationic lipids facilitate the delivery of mRNA vaccines, phospholipids, cholesterol, and lipid-anchored polyethylene glycol (PEG) are three other main lipid components required to ensure particle stability, delivery, and biodistribution (Reichmuth et al., 2016). Phospholipids are responsible for formation and disruption of the lipid bilayer for endosomal escape, sometimes having polymorphic features to promote a lamellar to hexagonal phase transition in the endosome (Reichmuth et al., 2016). Cholesterol modulates membrane rigidity and contributes to the transfection of cells where higher cholesterol content results in a lower transition temperature to aid lamellar phase transitions. Transitions to the hexagonal phase are necessary for the release of the mRNA from the LNP and its translocation across the endosomal membrane (Reichmuth et al., 2016). Lipid-anchored PEGs are preferentially deposited on the LNP surface and serves to sterically stabilize the LNP, acting as a barrier to reduce nonspecific binding to proteins. The PEGs that cover the LNPs influence its properties and is thus tailored according to its purpose. For example, high PEG content reduces cellular uptake and membrane interaction by circulating in the blood for longer (Reichmuth et al., 2016). Furthermore, LNP’s can have specific targeting sequences, antigens, signaling factors, and adjuvants added to its surface for specific applications.
Lipid nanoparticles designed for BNT162B2
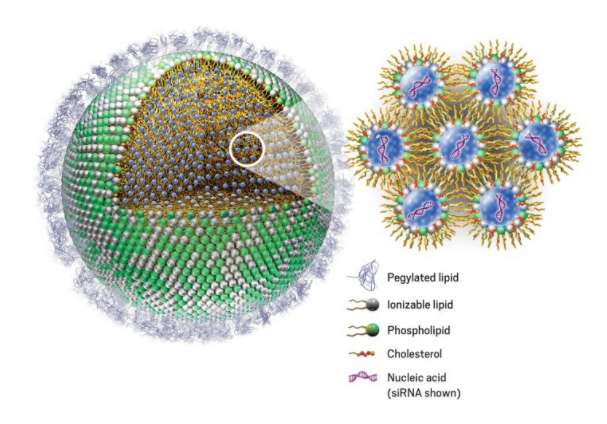
Figure 8: A lipid nanoparticle and the main components that make it up (Genevant Sciences).
For the BNT162b2 vaccine, the LNP formulation applied for improved mRNA delivery consisted of the ionizable/cationic lipid ALC-0315 (licensed from Acuitas Therapeutics) with quaternary amines that encapsulated the polyanionic mRNA; the helper lipid 1,2-distearoyl-snglycero-3-phosphocholine (DSPC) which resembles lipids in the cell membrane; cholesterol to stabilize the LNP’s lipid bilayer; and the PEG-lipid (PEG2000-DMA) to provide the nanoparticle a hydrating layer, improve colloidal stability, and reduce protein absorption (Granados-Riveron & Aquino-Jarquin, 2021).
Recent LNPs now represent the most advanced nucleic acid carrier packaging, protection, and efficient in-vivio delivery of mRNA. Clinical trials have demonstrated that lipid nanoparticles protect the mRNA from enzymatic degradation and enhance cellular uptake and expression to a maximum of 1000-fold compared to naked exogenous mRNA that is administered to animal models (Granados-Riveron & Aquino-Jarquin, 2021).
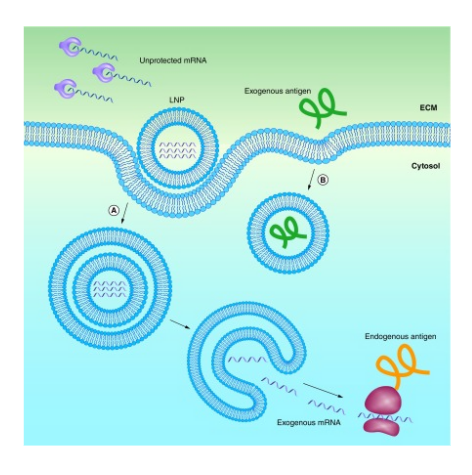
Figure 9: Endocytic pathway for a lipid nanoparticle and its endosomal escape and release of exogenous mRNA compared to an exogenous antigen trapped in an endosome by lacking a delivery system ((Reichmuth et al., 2016)).
Comparing the Delta and Omicron Variant against the Vaccine
The delta variant contains several notable mutations responsible for its increased infectivity and antibody escape. The first pair of altered residues are the D950N and P681R mutations located on the trimer interface which regulates spike protein dynamics and facilitate improved cell fusion and entry (Planas et al., 2021). Next, mutations relevant to antibody neutralization cluster in the NTD, with a deletion at 156-157 and mutations at G158R and T19R. These are positioned at the NTD “supersite” that is targeted by anti-NTD neutralizing antibodies (Planas et al., 2021). Furthermore, L452R and T478K are two RBD mutations found at the periphery of the ACE2-binding surface which impairs antibody neutralization. The T478K altered residue is unique to the delta variant and is near the E484K mutations that is found on the omicron variant and facilitates antibody escape. It is also within the epitope region for potent class 1 neutralizing monoclonal antibodies (Planas et al., 2021).
Key mutations found in the omicron variant were revealed to be the dominant epitopes in the RBD. While containing the same T478K mutation as the delta variant, it also contains a E484A mutation that confers the greatest impact on binding and neutralization (Greaney et al., 2021). This is speculated to be a result of the heavy-chain germline genes (IGHV3-53, and IGHV3-66 ) that are commonly utilized by RBD antibodies. Thus, E484K mutations can result in a greater than 10-fold reduction in polyclonal plasma neutralization. However, E484K mutations exhibit mild beneficial effects on receptor-binding affinity (Greaney et al., 2021). Mutations are also found on another major epitope formed by the residues 443-450 that make up a loop in the RBD’s RBM which greatly impact plasma antibody neutralization (Greaney et al., 2021). Furthermore, It was also reported that the omicron variant was able to evade neutralization from single BNT162b2 vaccinated individuals with a 12-44 fold higher efficiency than the delta variant (Hoffmann et al., 2021). However, Pfizer reported that their third dose imparts a 25-fold increase in neutralizing antibody titers compared to two doses against Omicron, while titers after the booster dose are comparable to titers seen against the wild type. Moreover, 80% of the epitopes recognized by cd8 t-cells are not affected, suggesting that two doses may still protect against severe disease (“Pfizer and BioNTech Provide Update on Omicron Variant | Pfizer,” n.d.). It is also speculated that the increased neutralizing response following the booster shot is a result of ongoing affinity maturation following initial vaccination or SARS-CoV-2 infection (Gruell et al., 2022).
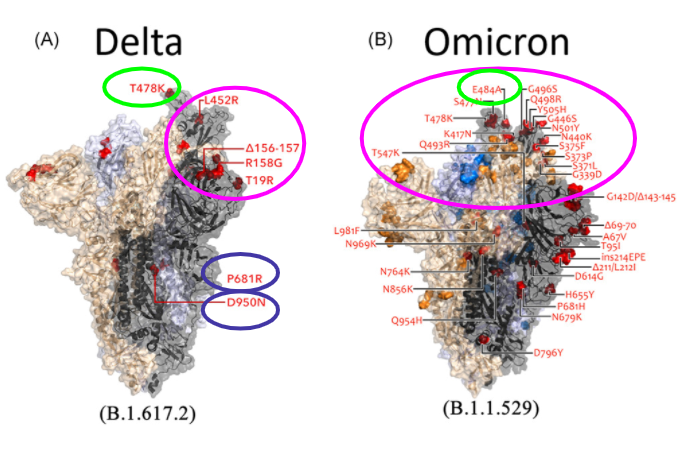
Figure 10: Key mutations of the delta and omicron variant. The blue circle represents mutations affecting infectivity. The pink circle represents the RBD and its NTD mutations responsible for majority of antibody escape. The green highlights the critical epitopes responsible for huge reductions in neutralizing antibodies. (Kumar, Thambiraja, Karuppanan, & Subramaniam, 2021).
Advantages
The current mRNA vaccines possess a multitude of advantages that make it preferable for future applications. When compared to traditional live-attenuated and subunit vaccines, it is able to induce both a cellular and humoral response while avoiding the risks of attenuated viruses having the rare occurrence of reverting to its pathogenic form for immunocompromised individuals. Therefore, mRNA vaccines possess the advantages of both subunit and live-attenuated vaccines without the associated risks (Reichmuth et al., 2016). Furthermore, mRNA vaccines are easier to deliver because they do not need to cross the nuclear membrane due to the cytosol being its target location. This removes the risk of integration or alteration of the host genome. The encoded mRNA transcript is also transiently expressed until its degradation, presenting only the minimal genetic information within the cell. The ability to encode multiple proteins with vastly different properties also make this vaccine extremely versatile with the ability to deliver a variety of antigens, modulators, and cell-signaling factors within a single molecule. Moreover, mRNA exhibits self-adjuvating properties by binding to pattern-recognition receptors such as TLR-7 that can promote cellular immunity. Finally, mRNA synthesis and purification is fast, easy, and low cost when compared to other vaccines, allowing for large scale production (Reichmuth et al., 2016).
Introduction to The Immunological Response of The Pfizer mRNA Vaccine
In order for a vaccine to be deemed effective, an immune response must be elicited such that immunological memory allows for the adaptive immune system to react towards a particular antigen (Sallusto et al., 2010). Upon vaccination, it is crucial for interplay between the innate and adaptive immune system to work together in order to provide long-term protection, and furthermore prevent the spread of infectious disease among individuals (Clem, 2011). Various adaptive immune cells such as CD4+ T Cells, CD8+ T Cells, Plasma B Cells, and Memory B cells have been studied in intensive detail for the BNT162b2 (common name: Pfizer mRNA vaccine) against SARS-CoV-2 (Samanovic et al., 2021).
Upon administration of the BNT162b2 mRNA vaccine, the mRNA residing in the lipid nanoparticle enters the host cell, and uses the host cell ribosome to translate a modified SARS-CoV-2 spike protein (Park et al., 2021). Some of the translated SARS-CoV-2 spike protein then encounters a proteasome to degrade the antigen into smaller peptide units (Park et al., 2021). These small degraded SARS-CoV-2 spike peptides are then taken up by either MHC Class I or MHC Class II, and displayed on the cell surface (Park et al., 2021). It is only when the MHC Class I or MHC Class II encounters a CD8+ T Cell or CD4+ T Cell, respectively, that triggers an immune cascade (Park et al., 2021).
Cytotoxic CD8+ T Cells
Cytotoxic CD8+ T Cells are adaptive immune cells that increase in concentration and activity upon BNT162b2 administration (Samanovic et al., 2021). Cytotoxic CD8+ T Cells are equipped with a T Cell Receptor (TCR) that is specific towards a certain MHC Class I and antigen pairing (Parham, 2021). MHC Class I is present in all nucleated cells (Parham, 2021). In the case of the BNT162b2 vaccine and SARS-CoV-2, the antigen that is present on the MHC Class I cell surface is the degraded SARS-CoV-2 spike protein (Park et al., 2021). After a match occurs between the TCR and it’s appropriate MHC Class I, the Cytotoxic CD8+ T Cell secretes various cytokines and cytotoxins to promote apoptosis in the infected cell (Parham, 2021). This is known as cell-mediated immunity, and the cytotoxic CD8+ T Cell is known as an effector CD8+ T Cell (Parham, 2021). Figure 11 illustrates the mechanism in which cytotoxic CD8+ T cells induce apoptosis in its cognate cell. Once the Cytotoxic CD8+ T Cell has been activated with the SARS-CoV-2 spike protein, a phenomenon known as clonal expansion occurs (Clem, 2011). Clonal expansion of cytotoxic CD8+ T cells increases the concentration of effector cytotoxic CD8+ T cells in the human body, as well as creating memory CD8+ T Cells (Clem, 2011). One theory proposed by von Essen et al. (2012) suggest that functional avidity maturation occurs at the clonal T Cell level, thus, stating that only those with a high avidity towards its antigen will be predominant within the clonal population.
After clearance of the SARS-CoV-2 antigen, a subset of the effector CD8+ T Cell become memory CD8+ T cells as a result of the BNT162b2 vaccine (Rha & Shin, 2021). These memory CD8+ cytotoxic T cells may either circulate the body or reside in resident tissues (Parham, 2021; Rha & Shin, 2021). According to the functional avidity maturation model stated by von Essen et al. (2012), memory CD8+ T Cells have a higher avidity towards the SARS-CoV-2 spike protein than a naive T cell (Samanovic et al., 2021). Interestingly, a study by Szabo et al. (2021) discovered that a majority of the cytotoxic CD8+ memory T cells reside in the airway of those administered BNT162b2. This provides enhanced protection as the SARS-CoV-2 virus infects the respiratory tract (Zhang et al., 2020). Having resident memory T cells within the respiratory pathway will provide immediate protection against SARS-CoV-2 upon reinfection (Parham, 2021). Overall, memory CD8+ T Cells promote immunological memory because their TCR has an increased affinity towards the SARS-CoV-2 spike protein and can provide immediate protection towards the antigen, should it be presented in the future (Parham, 2021; Samanovic et al., 2021; Rha & Shin, 2021).
Samanovic et al. (2021) concluded that CD8+ cytotoxic T cells activity and concentration increased significantly after each subsequent dose of BNT162b2. Samanovic et al. (2021) discovered that individuals who received a dose of BNT162b2 had an increase in Ki67+CD38+CD8+ T Cells, compared to those that did not have a dose of BNT162b2. Furthermore, Samanovic et al. (2021) found that after each subsequent dose of BNT162b2, there was an increase in SARS-CoV-2 specific CD8+ cytotoxic T cells. These two findings confirm that clonal expansion and functional avidity maturation has occurred to provide enhanced and prolonged protection against SARS-CoV-2 (von Essen et al., 2012; Clem, 2011). Finally, Samanovic et al. (2021) discovered that there was an increase in Granzyme B, TFN and IFN-y activity. An increase in these cytokines and cytotoxins suggest that infected cells are being eliminated, and that cell-mediated immunity is effective following BNT162b2 administration (Parham, 2021; Samanovic et al., 2021).
Germinal Centers
A hallmark feature of vaccines is to induce immunological memory towards a specific antigen (Plotkin, 2020). A mechanism that vaccines often use to induce immunological memory is through the class switching and affinity maturation of B Cells (Plotkin, 2020). These two processes both occur in the germinal center of a secondary lymphoid organ, such as the lymph node (Parham, 2021). In particular, a germinal center is divided into a dark and light zone (Parham, 2021). It is the dark zone that contains naive B cells, and the light zone that contains follicular dendritic cells (Parham, 2021). Together, the naive B cells, follicular dendritic cells and T follicular helper cells promote clonal expansion of naive B cells, somatic hypermutation, class switching and enhancing B cell affinity (Parham, 2021). Figure 12 illustrates the structure of the germinal center. Samanovic et al. (2021) discovered that upon administration of BNT162b2, there was an increase in germinal center formation as well as germinal center activity. Another study by Laidlaw & Ellebedy (2021) also found that germinal center B cell responses were still present in the draining lymph node even 6 months after administration. As the germinal center is crucial for creating long-lasting plasma B cells, and high affinity memory B cells, an increase in germinal centers is thereby correlated with increasing immunological memory (Samanovic et al., 2021; Laidlaw & Ellebedy 2021).
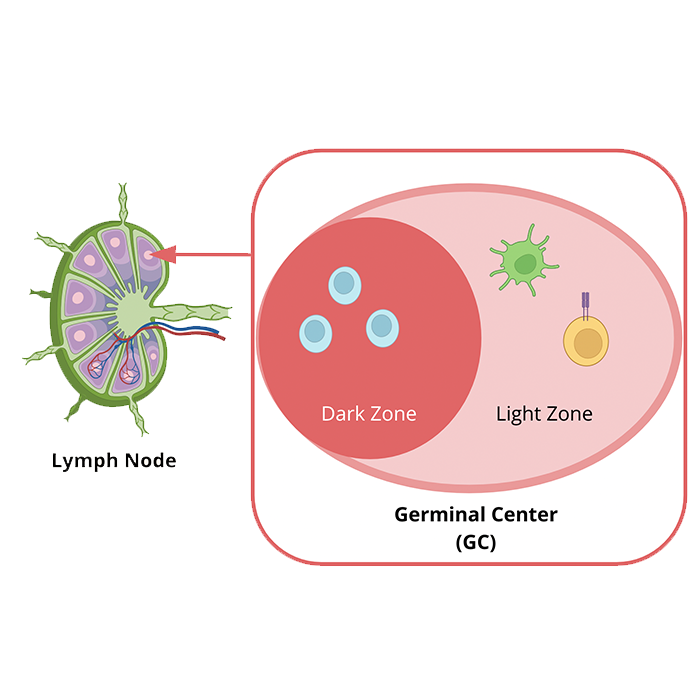
Figure 12: Magnified overview of the germinal center located in the lymph node. Light blue cells in the dark zone depict naive B cells, the yellow cell with a purple receptor represents a T CD4+ Follicular Helper cell, and the green cell represents a follicular dendritic cell. Image created by (BioRender, 2022), and information obtained from (Parham, 2021).
Perhaps, the increase in germinal center activity directly corresponds with our protection against COVID-19 variants (Laidlaw & Ellebedy, 2021). It is currently known that variants such as alpha, beta and omicron result from mutations within the SARS-CoV-2 genome (Zhang et al., 2020). As the dark and light zone of the germinal center are responsible for somatic hypermutation and enhancing B cell affinity, respectively (Parham, 2021), a heightened germinal center activity will evolve the current B cell response to produce high-affinity B cells towards the SARS-CoV-2 variants (Laidlaw & Ellebedy, 2021; Parham, 2021).
CD4+ Follicular T Cell and B Cells
In particular, there is a subset of CD4+ T Cells located in the the light zone of the germinal center known as T Follicular Helper Cells (Parham, 2021). These T Follicular Helper Cells increase in concentration after each subsequent dose of BNT162b2 (Samanovic et al., 2021). It is the role of the T Follicular Helper Cell to prime a naive B cell, and convert it into a plasma B cell or a memory B cell Samanovic et al. (2021). An increase in T Follicular Helper Cell concentration corresponds to a higher amount of naive B cells being primed to promote immunological memory (Parham, 2021; Samanovic et al., 2021). This was evidently found in the research study by Samanovic et al. (2021). By measuring CD21 and CD24 expression, Samanovic et al. (2020) noticed that both CD21 and CD24 expression decreased after each dose of BNT162b2. A decrease in CD21 and CD24 represents the presence of long-living plasma B cells and high affinity memory B cells, respectively (Samanovic et al., 2021). This again proves that BNT162b2 promotes immunological memory by providing long-lasting plasma B cells that can produce antibodies to neutralize the SARS-CoV-2 antigen (Samanovic et al., 2021). Similarly, high affinity memory B cells can bind to the SARS-CoV-2 antigen effectively and circulate around the body for a prolonged period of time (Laidlaw & Ellebedy, 2021).
Plasma B Cells and memory B cells have the ability to secrete antibodies to neutralize the SARS-CoV-2 antigen (Parham, 2021). Particularly, 2 antibodies the two antibodies that increased in concentration following each dose of BNT162b2 were: IgG and IgA antibodies (Samanovic et al., 2021). Figure 13 displays the increase in concentration of IgG and IgA antibodies after the 1st and 2nd dose of BNT162b2. Both IgG and IgA function to neutralize the SARS-CoV-2 antigen, however, IgG is more abundant in the tissue and blood (Wisnewski et al., 2021). In comparison, IgA is in a greater abundance within mucosal surfaces (Wisnewski et al., 2021). The higher antibody concentration following each dose of BNT162b2 indicate that immunity against SARS-CoV-2 is present (Samanovic et al., 2021).
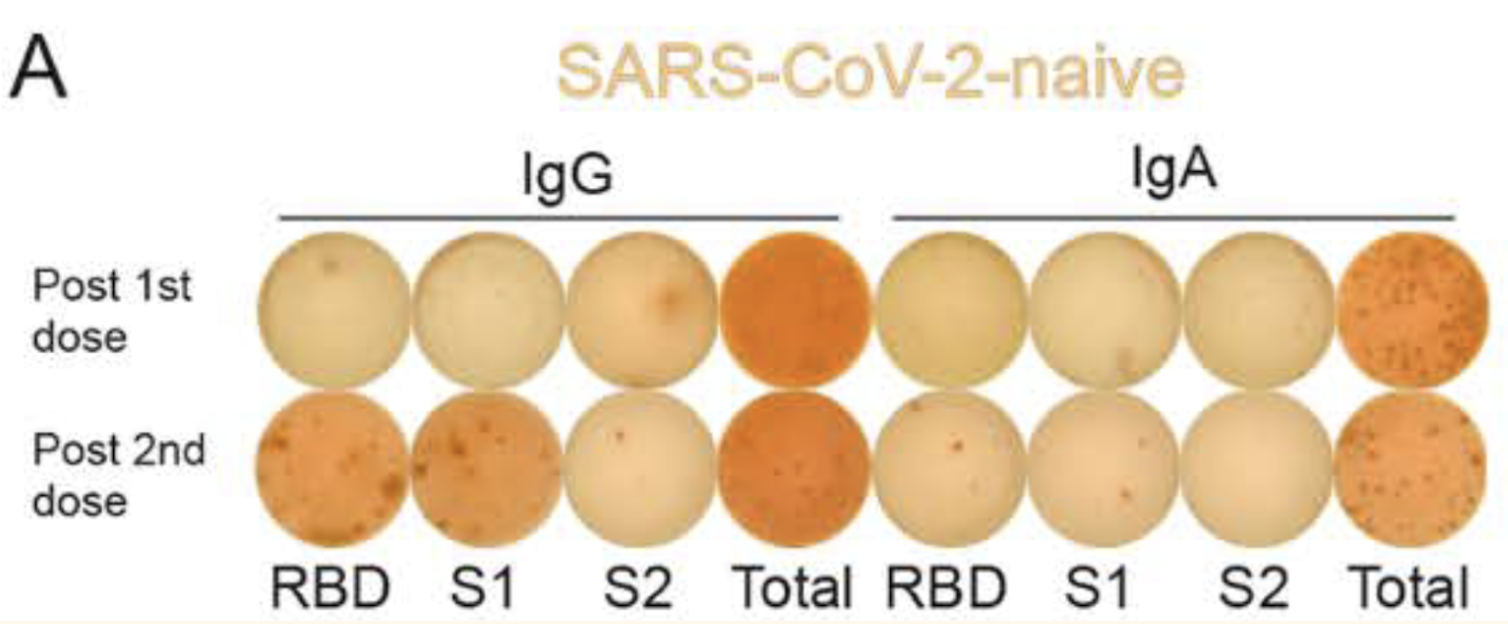
Figure 13: ELISpot displaying the concentration of IgG and IgA antibodies 10 days following the 1st and 2nd dose of BNT162b2. All subjects in this experiment are adults. (image adapted from: Samanovic et al., 2021)
Impact of Covid Vaccines
Covid-19 Vaccines have helped to improve public health overall (Tenforde et al., 2021). The benefits of the vaccine can be seen in the association with vaccination status and hospitalization (Tenforde et al., 2021). In Ontario, unvaccinated patients make up the majority of the cases, as well as nearly all of the hospitalized and ICU patients, as seen in Figure 14 (Ontario Covid-19 Science Advisory Table, 2022). As cases are generally lower in the proportion of the population that is vaccinated, there is a correlation between vaccination and protection against Covid-19 (Tenforde et al., 2021)
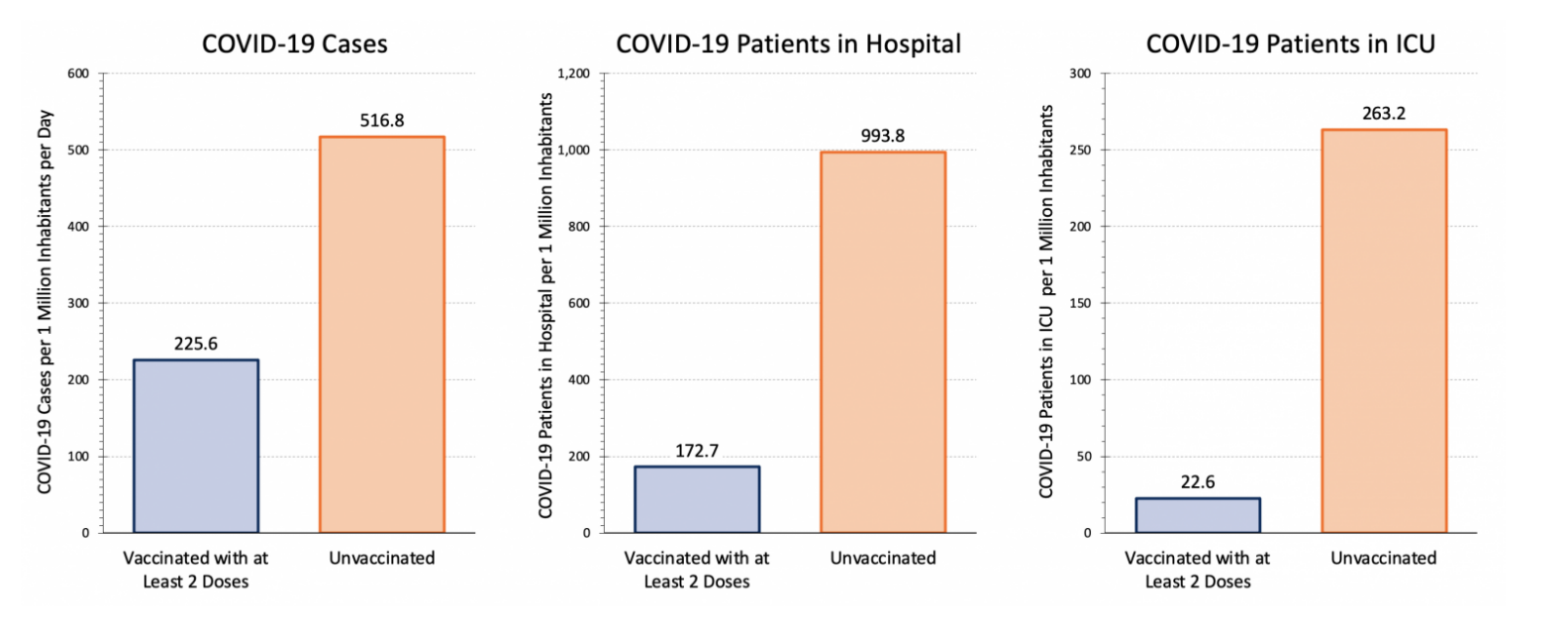
Figure 14: Covid-19 case numbers in Ontario on January 31, 2022 in unvaccinated and vaccinated groups (image adapted from: Ontario Covid-19 Science Advisory Table, 2022).
However, there are still individuals who have received at least two doses of the vaccine who develop Covid-19, which can be seen clearly in Figure 15 (Ontario Covid-19 Science Advisory Table, 2022). Especially during late December and early January, there are many cases associated with the Omnicron variant in individuals who are vaccinated (Ontario Covid-19 Science Advisory Table, 2022). Despite this, patients who are hospitalized with Covid-19 are at a lower risk of death or invasive mechanical ventilation if they are also vaccinated (Tendforde et al., 2021). This can also be seen in Figure 15, as there are relatively few patients in the hospital and ICU who are vaccinated compared to the staggering majority who are not (Ontario Covid-19 Science Advisory Table, 2022). Therefore, vaccination prevents infection to a degree, but also leads to a lessened disease severity among individuals who still develop Covid-19 despite being vaccinated (Tenforde et al., 2021).
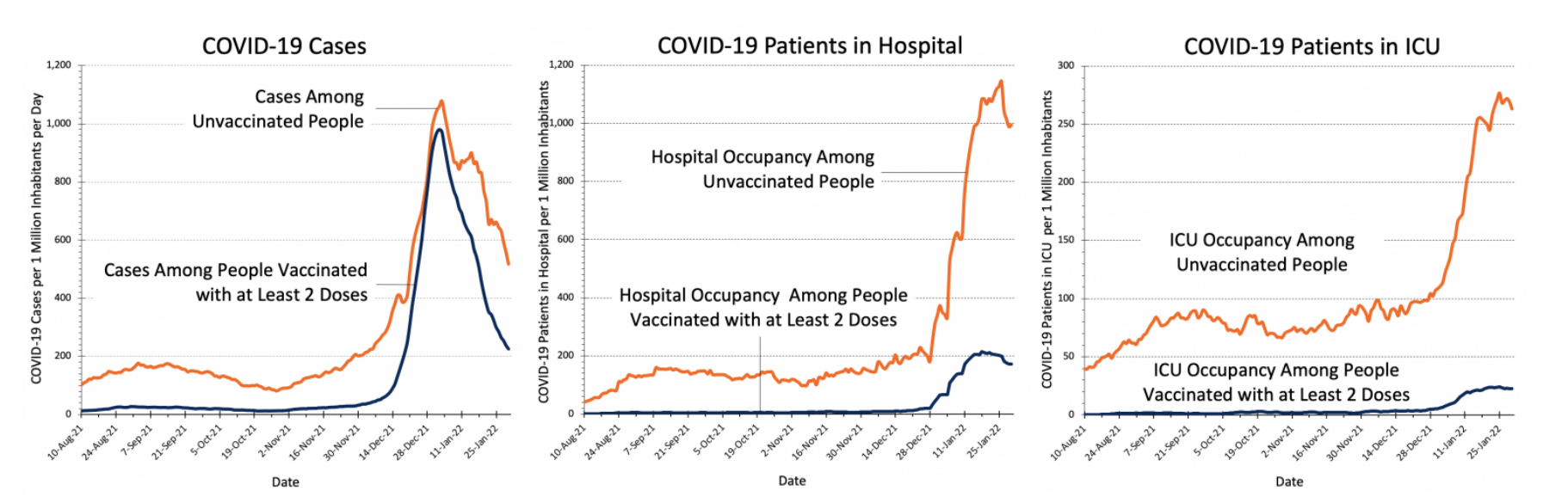
Figure 15: Covid-19 case numbers in Ontario from August 2021 to January 2022 in vaccinated and unvaccinated groups (image adapted from: Ontario Covid-19 Science Advisory Table, 2022).
Up-to-date data on Covid-19 data in Ontario can be found on the Science Table Ontario Dashboard.
Conclusion
Although there have been many advancements to create SARS-CoV-2 vaccines, there are still unknowns about it’s efficacy (Samanovic et al., 2021). Both vaccination and prior infection of SARS-CoV-2 have been associated with a degree of protection against reinfection, however, it is not known how these two factors may influence each other (Samanovic et al., 2021). Additionally, the duration of protection, the full spectrum of immune responses to these vaccines, and whether or not these vaccines induce immunological memory are still in question (Samanovic et al., 2021).
Having a grasp of how vaccination with and without prior infection influences immune response would allow for more accurate health care (Samanovic et al., 2021). By understanding how vaccination and prior infection shapes immunological memory, the susceptibility of individuals with a broad range of experience can be better predicted (Samanovic et al., 2021). Additionally, this would allow for personalized vaccine strategies for booster shots to maintain immunological memory (Samanovic et al., 2021). In Figure 15, there is an increase in cases towards the end of 2021, which may be attributed to the Omicron variant, but could also be partially attributed to a lack of booster shots in certain individuals who do have two doses of the vaccine (Ontario Covid-19 Science Advisory Table, 2022) (Tenforde et al., 2021). Therefore, investigation into the effects and response generated by the vaccine and prior infection of SARS-CoV-2 could have great applications (Samanovic et al., 2021).
References
BioRender. (2022). Create Professional Science Figures in Minutes. BioRender. Retrieved January 29, 2022, from https://biorender.com/
Bollinger, R., & Ray, S. (2022, January 14). Covid variants: What you should know. COVID Variants: What You Should Know. Retrieved February 3, 2022, from https://www.hopkinsmedicine.org/health/conditions-and-diseases/coronavirus/a-new-strain-of-coronavirus-what-you-should-know
Centers for Disease Control and Prevention. (2021). Symptoms of COVID-19. Centers for Disease Control and Prevention. Retrieved February 3, 2022, from https://www.cdc.gov/coronavirus/2019-ncov/symptoms-testing/symptoms.html Clem A. S. (2011). Fundamentals of vaccine immunology. Journal of global infectious diseases, 3(1), 73–78. https://doi.org/10.4103/0974-777X.77299
Coronavirus disease (COVID-19): How is it transmitted? (2021, December 21). Coronavirus disease (covid-19): How is it transmitted? World Health Organization. Retrieved February 3, 2022, from https://www.who.int/emergencies/diseases/novel-coronavirus-2019/question-and-answers-hub/q-a-detail/coronavirus-disease-covid-19-how-is-it-transmitted
Dong, Y., Dai, T., Wei, Y., Zhang, L., Zheng, M., & Zhou, F. (2020). A systematic review of SARS-CoV-2 vaccine candidates. Signal Transduction and Targeted Therapy, 5(1). https://doi.org/10.1038/s41392-020-00352-y
Gavi. (2020). There are four types of COVID-19 vaccines: Here's how they work. Gavi. https://www.gavi.org/vaccineswork/there-are-four-types-covid-19-vaccines-heres-how-they-work
Granados-Riveron, J. T., & Aquino-Jarquin, G. (2021). Engineering of the current nucleoside-modified mRNA-LNP vaccines against SARS-CoV-2. Biomedicine & Pharmacotherapy, 142, 111953. https://doi.org/10.1016/J.BIOPHA.2021.111953
Greaney, A. J., Loes, A. N., Crawford, K. H. D., Starr, T. N., Malone, K. D., Chu, H. Y., & Bloom, J. D. (2021). Comprehensive mapping of mutations in the SARS-CoV-2 receptor-binding domain that affect recognition by polyclonal human plasma antibodies. Cell Host & Microbe, 29(3), 463. https://doi.org/10.1016/J.CHOM.2021.02.003
Gruell, H., Vanshylla, K., Tober-Lau, P., Hillus, D., Schommers, P., Lehmann, C., … Klein, F. (2022). mRNA booster immunization elicits potent neutralizing serum activity against the SARS-CoV-2 Omicron variant. Nature Medicine 2022, 1–4. https://doi.org/10.1038/s41591-021-01676-0
Heinz, F. X., & Stiasny, K. (2021). Distinguishing features of current COVID-19 vaccines: knowns and unknowns of antigen presentation and modes of action. NPJ vaccines, 6(1), 104. https://doi.org/10.1038/s41541-021-00369-6
Hou, X., Zaks, T., Langer, R., & Dong, Y. (2021). Lipid nanoparticles for mRNA delivery. Nature reviews. Materials, 1–17. Advance online publication. https://doi.org/10.1038/s41578-021-00358-0
Hoffmann, M., Krüger, N., Schulz, S., Cossmann, A., Rocha, C., Kempf, A., … Pöhlmann, S. (2021). The Omicron variant is highly resistant against antibody-mediated neutralization: Implications for control of the COVID-19 pandemic. Cell. https://doi.org/10.1016/J.CELL.2021.12.032
Naming the coronavirus disease (COVID-19) and the virus that causes it. (n.d.). Naming the coronavirus disease (COVID-19) and the virus that causes it. World Health Organization. Retrieved February 3, 2022, from https://www.who.int/emergencies/diseases/novel-coronavirus-2019/technical-guidance/naming-the-coronavirus-disease-(covid-2019)-and-the-virus-that-causes-it
Juni, P., da Costa, B. R., Maltsev, A., G M, Perkhun, A., Yan, S., & N S. (2021). Ontario dashboard. Ontario COVID-19 Science Advisory Table. Retrieved February 2, 2022, from https://covid19-sciencetable.ca/ontario-dashboard/
Kang, H. M., Choi, E. H., & Kim, Y. J. (2021). Updates on the coronavirus disease 2019 vaccine and consideration in children. Clinical and Experimental Pediatrics. https://doi.org/10.3345/cep.2021.00696
Krammer, F. (2020). SARS-CoV-2 vaccines in development. Nature, 586(7830), 516–527. https://doi.org/10.1038/s41586-020-2798-3
Kumar, S., Thambiraja, T. S., Karuppanan, K., & Subramaniam, G. (2021). Omicron and Delta variant of SARS-CoV-2: A comparative computational study of spike protein. Journal of Medical Virology, 1–9. https://doi.org/10.1002/JMV.27526
Ma, Q., Liu, J., Liu, Q., Kang, L., Liu, R., & Jing, W. et al. (2021). Global Percentage of Asymptomatic SARS-CoV-2 Infections Among the Tested Population and Individuals With Confirmed COVID-19 Diagnosis. JAMA Network Open, 4(12), e2137257. doi: 10.1001/jamanetworkopen.2021.37257
Parham, P. (2021). The Immune System (5th ed.). W. W. Norton & Company. Park, J. W., Lagniton, P., Liu, Y., & Xu, R. H. (2021). mRNA vaccines for COVID-19: what, why and how. International journal of biological sciences, 17(6), 1446–1460. https://doi.org/10.7150/ijbs.59233
Pfizer and BioNTech Provide Update on Omicron Variant | Pfizer. (n.d.). Retrieved February 3, 2022, from https://www.pfizer.com/news/press-release/press-release-detail/pfizer-and-biontech-provide-update-omicron-variant
Planas, D., Veyer, D., Baidaliuk, A., Staropoli, I., Guivel-Benhassine, F., Rajah, M. M., … Schwartz, O. (2021). Reduced sensitivity of SARS-CoV-2 variant Delta to antibody neutralization. Nature 2021 596:7871, 596(7871), 276–280. https://doi.org/10.1038/s41586-021-03777-9 Plotkin S. A. (2010). Correlates of protection induced by vaccination. Clinical and vaccine immunology : CVI, 17(7), 1055–1065. https://doi.org/10.1128/CVI.00131-10
Prates-Syed, W. A., Chaves, L. C. S., Crema, K. P., Vuitika, L., Lira, A., Côrtes, N., Kersten, V., Guimarães, F. E. G., Sadraeian, M., Barroso Da Silva, F. L., Cabral-Marques, O., Barbuto, J. A. M., Russo, M., Câmara, N. O. S., & Cabral-Miranda, G. (2021). VLP-Based COVID-19 Vaccines: An Adaptable Technology against the Threat of New Variants. Vaccines, 9(12), 1409. https://doi.org/10.3390/vaccines9121409
Reichmuth, A. M., Oberli, M. A., Jeklenec, A., Langer, R., & Blankschtein, D. (2016). mRNA vaccine delivery using lipid nanoparticles. Therapeutic Delivery, 7(5), 319. https://doi.org/10.4155/TDE-2016-0006
Rha, M. S., & Shin, E. C. (2021). Activation or exhaustion of CD8+ T cells in patients with COVID-19. Cellular & molecular immunology, 18(10), 2325–2333. https://doi.org/10.1038/s41423-021-00750-4
Sallusto, F., Lanzavecchia, A., Araki, K., & Ahmed, R. (2010). From vaccines to memory and back. Immunity, 33(4), 451–463. https://doi.org/10.1016/j.immuni.2010.10.008
Samanovic, M. I., Cornelius, A. R., Gray-Gaillard, S. L., Allen, J. R., Karmacharya, T., Wilson, J. P., Wesley Hyman, S., Tuen, M., Koralov, S. B., Mulligan, M. J., & Sedaghat Herati, R. (2021). Robust immune responses are observed after one dose of BNT162b2 mRNA vaccine dose in SARS-CoV-2 experienced individuals. Science translational medicine, eabi8961. Advance online publication. Schoeman, D., & Fielding, B. (2019). Coronavirus envelope protein: current knowledge. Virology Journal, 16(1). doi: 10.1186/s12985-019-1182-0
Shukla, D. (2021, September 28). DNA vs. mRNA vaccines: Similarities and differences. Medical News Today. https://www.medicalnewstoday.com/articles/dna-vs-mrna-vaccines-similarities-and-differences
Syed, A., Taha, T., Tabata, T., Chen, I., Ciling, A., & Khalid, M. et al. (2021). Rapid assessment of SARS-CoV-2–evolved variants using virus-like particles. Science, 374(6575), 1626-1632. doi: 10.1126/science.abl6184
Szabo, P. A., Dogra, P., Gray, J. I., Wells, S. B., Connors, T. J., Weisberg, S. P., Krupska, I., Matsumoto, R., Poon, M., Idzikowski, E., Morris, S. E., Pasin, C., Yates, A. J., Ku, A., Chait, M., Davis-Porada, J., Guo, X. V., Zhou, J., Steinle, M., Mackay, S., … Farber, D. L. (2021). Longitudinal profiling of respiratory and systemic immune responses reveals myeloid cell-driven lung inflammation in severe COVID-19. Immunity, 54(4), 797–814.e6. https://doi.org/10.1016/j.immuni.2021.03.005
Tenforde, M. W., Self, W. H., Adams, K., Gaglani, M., Ginde, A. A., McNeal, T., Ghamande, S., Douin, D. J., Talbot, H. K., Casey, J. D., Mohr, N. M., Zepeski, A., Shapiro, N. I., Gibbs, K. W., Files, D. C., Hager, D. N., Shehu, A., Prekker, M. E., Erickson, H. L., … Patel, M. M. (2021). Association between mrna vaccination and COVID-19 hospitalization and disease severity. JAMA, 326(20), 2043. https://doi.org/10.1001/jama.2021.19499
Thomas, S. (2020). The Structure of the Membrane Protein of SARS-CoV-2 Resembles the Sugar Transporter SemiSWEET. Pathogens And Immunity, 5(1), 342. doi: 10.20411/pai.v5i1.377
Understanding SARS-CoV-2 and the drugs that might lessen its power – The Economist | Medical School. (2022). Retrieved 3 February 2022, from https://www.medicalschool.tv/anatomy/understanding-sars-cov-2-and-the-drugs-that-might-lessen-its-power-the-economist/
Viganò, S., Utzschneider, D. T., Perreau, M., Pantaleo, G., Zehn, D., & Harari, A. (2012). Functional avidity: a measure to predict the efficacy of effector T cells?. Clinical & developmental immunology, 2012, 153863. https://doi.org/10.1155/2012/153863 von Essen, M. R., Kongsbak, M., & Geisler, C. (2012). Mechanisms behind functional avidity maturation in T cells. Clinical & developmental immunology, 2012, 163453. https://doi.org/10.1155/2012/163453
Wang, M., Zhao, R., Gao, L., Gao, X., Wang, D., & Cao, J. (2020). SARS-CoV-2: Structure, Biology, and Structure-Based Therapeutics Development. Frontiers In Cellular And Infection Microbiology, 10. doi: 10.3389/fcimb.2020.587269
WHO. (2021). The different types of COVID-19 vaccines. World Health Organization. https://www.who.int/news-room/feature-stories/detail/the-race-for-a-covid-19-vaccine-explained
WHO Coronavirus (COVID-19) Dashboard. (2022). Retrieved 3 February 2022, from https://covid19.who.int/ WHO Timeline - COVID-19. (2020, April 27). Archived: Who timeline - covid-19. World Health Organization. Retrieved February 3, 2022, from https://www.who.int/news/item/27-04-2020-who-timeline---covid-19
Wisnewski, A. V., Campillo Luna, J., & Redlich, C. A. (2021). Human IgG and IgA responses to COVID-19 mRNA vaccines. PloS one, 16(6), e0249499. https://doi.org/10.1371/journal.pone.0249499
Zhang, Y., Geng, X., Tan, Y., Li, Q., Xu, C., Xu, J., Hao, L., Zeng, Z., Luo, X., Liu, F., & Wang, H. (2020). New understanding of the damage of SARS-CoV-2 infection outside the respiratory system. Biomedicine & pharmacotherapy = Biomedecine & pharmacotherapie, 127, 110195. https://doi.org/10.1016/j.biopha.2020.110195