Table of Contents
Structural basis for unidirectional export of lipopolysaccharide to the cell surface
Tristan W. Owens, Rebecca J. Taylor, Karanbir S. Pahil, Blake R. Bertani, Natividad Ruiz, Andrew C. Kruse & Daniel Kahne | Nature volume 567, pages 550–553 (2019)
Introduction to the Paper
The following article by Owens et al. concerns gram-negative bacteria. Gram-negative bacteria are an abundant and infectious group of microorganisms that contain innate resistance to many antimicrobials due to the additional outer membrane. In contrast, gram-positive bacteria lack an outer membrane which makes them more susceptible to antimicrobial treatments. Lipopolysaccharides (LPS) is an essential component of the outer membrane and has garnered attention as a potential drug target. The structure and synthesis of LPS has been elucidated. LPS is synthesized endogenously by gram-negative bacteria in the inner membrane and then transported onto the surface of the outer membrane. A better understanding of the components and mechanisms of the LPS transport system is required. Owens et al. addressed this concern by creating two models of LPS transport in two species of bacteria (Vibrio cholerae and Enterobacter cloacae). Their research solidified aspects of LPS transport and also identified a protein gate mechanism that allows unidirectional movement of LPS molecules from the inner membrane to the outer membrane. In this first Wiki page, a background on gram-negative bacteria, LPS structure and synthesis, and a brief introduction to LPS transport will be presented.
Introduction to Gram-Negative Bacteria
Bacteria are prokaryotic organisms that make up one of the three domains of life. They are unicellular and carry their genetic information in a double-stranded circular molecule of DNA. The structure among the different species stays fairly consistent with a cell cytoplasm that contains ribosomes, a cell membrane, and in all species but one, a cell wall. Outside the cell envelope, some types of bacteria have other motor-based structures such as capsules, flagella, or pili. Bacteria reproduce using binary fission and under the proper growth conditions, they can divide and multiply quite rapidly (1).
The Gram Stain
Bacteria are one of the most diverse life forms on earth, and may consist of more than one million species (2). One way to differentiate between species is by using the Gram staining method developed in 1882 by Hans Christian Gram (1). To do a Gram stain, you first apply the primary crystal violet stain, followed by the addition of a mordant, which is typically Gram’s iodine. After this rapid decolorization is done with ethanol, acetone or a mixture of both. Finally, the counterstain safranin is added. Bacteria are classified as either Gram-positive or Gram-negative based on the composition of their envelope, and how they are seen under a microscope after the stains have been administered (3).
Figure 1. The Gram staining protocol. This process was discovered by Hans Christian Gram, and uses four simple staining and washing steps to differentiate between different types of bacteria. Gram-negative bacteria (stained pink) have a diderm cell envelope with a thin layer of peptidoglycan in their periplasm. Gram-positive bacteria (stained violet) have a monoderm cell envelope with a thick layer of peptidoglycan. (Image created in BioRender.com).
Gram-Negative and Gram-Positive Bacteria
Gram-negative bacteria do not retain the crystal violet stain because they contain a lipid rich outer membrane, and this allows the alcohol decolourizing step to wash the crystal violet from the cells and the safranin then colours the bacteria red instead (4). Gram-positive bacteria will show up violet which gives a positive result for the Gram stain test5. Some bacteria are not classified as Gram-positive or Gram-negative, and these include the mycobacteria (1).
This test is considered a bit out-dated now, since it focuses too much on the specific phyla of bacteria Firmicutes and Proteobacteria, as represented by the model organisms Bacillis subtilis and Escherichia coli. Now, the better way to define species is by their cell envelope architecture, as either a monoderm or a diderm. Gram-positive bacteria have a monoderm structure, they have an inner membrane, but lack an outer membrane. Instead they are surrounded by thick layers of peptidoglycan. Gram-negative bacteria have a diderm structure, with an inner membrane surrounded by a thin peptidoglycan layer, which is all encompassed within an outer membrane (5).
Cell Envelope and Membrane Structure of Gram-Negative Bacteria
The Gram-negative cell envelope consists of two membranes, the inner and the outer, as well as a space in between called the periplasm which contains a thin peptidoglycan layer (4).
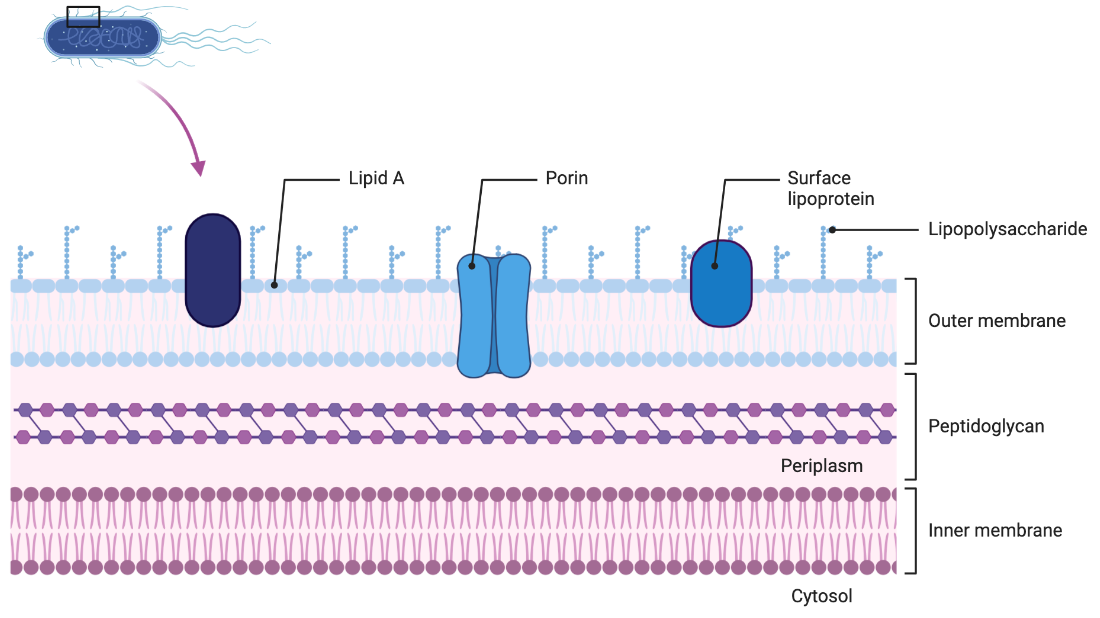
Figure 4. Gram-negative cell envelope structure. This diagram shows the structure of the cell envelope found in Gram-negative bacteria. There are three distinct layers consisting of the inner membrane, periplasm, and the outer membrane. Some unqiue features include a thin peptidoglycan cell wall in the periplasm, and lipopolysaccharides found on the outer leaflet of the outer membrane. (Image created in BioRender.com).
Inner Membrane
The inner membrane (IM) of Gram-negative bacteria includes a symmetric bilayer of glycerophospholipids (6). These molecules create a variety of sensors that are embedded within the IM, which are able to sense an environmental change from incoming stimuli (6). Lateral diffusion characterizes the IM and creates a specific flow of molecules throughout this component of Gram-negative bacteria (6). This membrane structure is connected to the outer membrane by membrane-spanning protein complexes through covalent and noncovalent protein linkages (6).
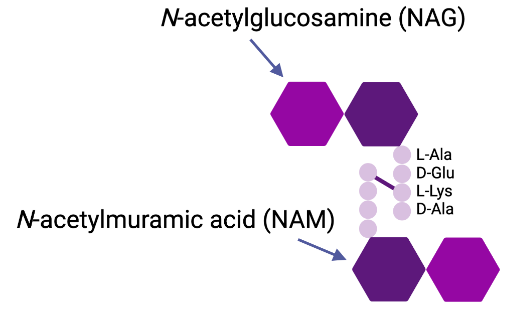
Figure 5: The Structure of Peptidoglycan. The backbone of peptidoglycan is composed of alternating units of N-acetylglucosamine and N-acetylmuramic acid which are linked together by glycosidic bonds. The attached peptide subunits contain four alternating L- and D-amino acids, and they are connected to the glycan stands by the lactyl groups of the N-acetylmuramic acid. (Image created in BioRender.com).
Periplasm
The periplasm is an aqueous cellular compartment that contains a thin peptidoglycan cell wall layer within Gram-negative bacteria (7). The peptidoglycan is responsible for determining the major shape in bacterial cells (7). Peptidoglycan is composed of repeating units of the N-acetyl glucosamine-N-acetyl muramic acid disaccharide, which are cross-linked by pentapeptide side chains (7). Enzyme or antibiotic agents capable of damaging the peptidoglycan layer can cause cell lysis, overcoming the turgor pressure of the cytoplasm (7). The periplasm is densely packed with proteins, making it more viscous than the cytoplasm (7). The periplasmic space is the area containing the periplasm, where it is characterized by a concentrated gel-like matrix (4). This area is a dynamic region between the two bilayer membranes that possess an array of macromolecules that represent the cell’s metabolic status (4).
Outer Membrane
The outer membrane (OM) of Gram-negative bacteria separates the external environment from the periplasm, and has the essential role of providing protection for the organism, while also allowing for efficient exchange of the materials and resources needed to sustain the life of the bacterium (4). The OM is an asymmetric bilayer made up of phospholipids in the inner leaflet and lipopolysaccharides (LPS) as well as phospholipids in the outer leaflet (4). The phospholipid composition of the inner leaflet of the OM is similar to that of the cytoplasmic membrane, with about 80% being made up of phosphatidylethanolamine, 15% phosphatidylglycerol and 5% cardiolipin (8). A typical LPS molecule consists of three parts, lipid A, a relatively short core oligosaccharide, and a distal polysaccharide called the O-antigen (8).
The highly hydrophobic phospholipid bilayer also contains pore-forming proteins called porins with specific size-exclusion properties, and together these allow for the OM to act as a selective barrier (8). The OM is much more porous than the inner membrane, with the degree of porosity varying between species (4). This being said, the barrier cannot be too porous or else the larger periplasmic constituents vital to the cell will not be retained (4). The permeability properties also have a major impact on the susceptibility of the bacteria to antibiotics which typically target intracellular processes (8). For example, small hydrophilic drugs, such as β-lactams, can use the porins to access the cell interior, and other hydrophobic drugs can diffuse across the lipid bilayer (8). LPS is exclusively found in the outer leaflet of the outer membrane, and it plays many important roles including maintaining the integrity of the outer-membrane permeability barrier and participating extensively in host–pathogen interplay (4). The importance of the OM barrier in relation to antibiotic efficacy can especially be seen through the existence of drug-resistant strains of bacteria. These mutant strains often have modifications in the lipid or protein composition of the OM (8).
There are two classes of proteins that exist in the OM, these are lipoproteins and β-barrel proteins (7). Lipoproteins contain an amino-terminal cysteine residue that is attached to lipid moieties that embed the lipoprotein in the inner leaflet of the OM (7). β-barrel proteins are transmembrane proteins, and they are often referred to as outer membrane proteins or OMPs (7). These OMPs are made up of β sheets that are wrapped into cylinders, and some examples include the porins OmpF and OmpC which function to allow the passive diffusion of small molecules across the OM (7).
LPS Overview
Lipopolysaccharides (LPS) are complex glycolipids produced by most gram-negative bacteria. They are anchored to the outer leaflet of the outer membrane (OM) and contribute to the asymmetric OM bilayer. LPS is an essential and well-conserved component of the OM and the life of bacteria that produce it. It first gained attention for its ability to trigger the host immune system by acting as an endotoxin. From there, other functions of LPS have been revealed. The biosynthesis and intracellular transport of LPS involves a unique class of proteins. Since LPS is only found in bacteria, components associated with LPS synthesis, transport, and function have become potential drug targets and are promising routes for antibiotic development (9).
LPS Structure
LPS is a large glycolipid composed of 3 structural domains: Lipid A, core oligosaccharide, and the O-antigen. The overall structure is generally conserved at the species and strain level, with the exception of the O-antigen (9).
Lipid A is the structural basis of the molecule that attaches to the outer leaflet of the outer membrane. It is a hydrophobic, hexaacylated, diphosphorylated glucosamine disaccharide. Since the Lipid A component is the most conserved region of LPS, it is typically recognized by host organisms as an endotoxin. However, variations between different species may lead to varying immune responses in different hosts (9).
The inner oligosaccharide core is a conserved structural element of LPS. It consists of two Kdo (3-deoxy-a-d-manno-oct-2-ulopyranosonic acid) residues, as well as several heptoses and phosphate groups. The outer core is more structurally diverse than the inner core and contains hexoses, such as glucose, galactose, and N-acetylglucosamine (GlcNAc groups)(9).
The O-antigen repeat is a highly variable region that protrudes onto the surface of the cell membrane. It is composed of repeating oligosaccharide units of 2-8 sugars, though total length of an O-antigen varies greatly and can go up to 40 repeat units. Research has shown that O-antigen may be involved in bacterial immune evasion tactics, such as avoiding activation of host complement systems. Further, its extensive variation between strain and species is a useful method for identifying and classifying bacteria, in a process known as O-serotyping (9).
LPS Function
LPS serves four main functions (9): 1) as a structural component of the OM, 2) as a permeability barrier, 3) as an endotoxin, and 4) as an identifier. LPS is anchored to the OM through its Lipid A component, whereas the core oligosaccharides and O-antigen are exposed on the exterior of the cell. The presence of LPS provides structural integrity which protects the bacteria from toxins, bile salts, and other environmental stressors. Additionally, LPS contains a net negative charge due to phosphate and carboxylate groups that are present throughout its structure. The overall negative charge of bacterial membranes differentiates it from mammalian membranes. Antimicrobials take advantage of this feature to specifically target bacterial cells (9).
Due to the OM, gram-negative bacteria have a survival advantage and greater resistance to antimicrobial compounds when compared to gram-positive bacteria. This is because LPS acts as a permeability barrier that excludes entry of small, hydrophobic molecules that would otherwise cross the phospholipid bilayers. LPS is an amphipathic compound with hydrophilic and hydrophobic components (9). The hydrophilic oligosaccharides and O-antigen prevent entry of hydrophobic molecules, whereas the hydrophobic acyl chains of Lipid A prevent entry of hydrophilic molecules. The effectiveness of the permeability barrier is dependent on how tightly LPS packs together. The packing of LPS is due to hydrophobic associations between the acyl chains of Lipid A. However, this is complicated by negatively charged phosphate groups on Lipid A. To minimize repulsion, divalent cations such as Magnesium (Mg2+) intercalates between LPS. Hydrophobic and polyionic interactions work together to ensure LPS is densely packed to create a strong barrier against harmful substances (9).
Thirdly, LPS functions as an endotoxin that is able to trigger host immune responses. During infection, hosts have evolved ways to recognize the most conserved regions of LPS, particularly the Lipid A component. LPS is recognized as a pathogen-associated molecular pattern (PAMP) using specialized receptors in the host. Toll-like receptor 4s (TLR4) are the primary receptors for Lipid A (9). Upon ligand binding, a cascade of events occur to ultimately produce inflammatory cytokines and interferons. Though Lipid A is the most conserved portion of LPS, diversity between bacterial species/strains exists and varying immune responses to an infection can occur. However, an arms race between bacteria and the host exists. Bacteria have evolved to evade host recognition by altering or masking components of LPS. The hexa-acylated and biphosphorylated structure of Lipid A is highly immunogenic (9). Some bacteria can produce a less-acylated Lipid which allows them to evade host immune cells (10). A longer O-antigen has also been observed to protect bacteria against the complement system. Longer O-antigen repeats interfere with the deposition of complements on bacterial surfaces, thus protecting bacteria from antibody-mediated responses and phagocytosis (11).
Lastly, LPS can be used to identify and classify bacteria in a procedure called O-serotyping. The O-antigen on LPS is highly variable and consists of repeating oligosaccharides that can be modified in a variety of ways. The unique sugar composition and the antibodies activated in response to these structures is how bacterial strains can be distinguished from one another. Bacteria within a serotype typically display the same type and number of surface antigens. O-serogroups for Escherichia coli, for example, are commonly used for identifying E.coli strains during epidemiological and phylogenetic studies (12).
LPS Synthesis
LPS Synthesis occurs primarily in the cytoplasm, with completed components being translocated to the periplasm for ligation. There are 3 main phases: Lipid A synthesis, oligosaccharide synthesis, and O-antigen synthesis. The synthesis of oligosaccharide is dependent upon completion of Lipid A synthesis. O-antigen synthesis is independent of the other two phases. A summary of the various steps are as follows (9):
Lipid A Synthesis
Lipid A synthesis begins with precursor molecule N-acetyl glucosamine attached to the nucleotide carrier molecule UDP-GlcNAc. There are 9 principle steps, including 6 acylations and 2 phosphorylations. The steps are as follows (9):
- UDP-GlcNAc is first acylated by LpxA.
- LpxC deacylation.
- LpxD acylation → UDP-2,3-diacylglucosamine
- LpxH removes the sugar nucleotide carrier → produces intermediate “Lipid X”
- LpxB adds Lipid X + UDP-2,3-diacylglucosamine (intermediate product of step #4) → tetraacylated glucosamine disaccharide (Lipid A disaccharide).
- Product of #5 is inserted into the inner leaflet of IM.
- LpxK phosphorylates Lipid A disaccharide at 4’ position → bi-phosphorylated lipid IVa
- LpxL acylates by adding lauroyl group
- LpxM acylates by adding myristoyl group (note: 8 & 9 are close together; LpxM can function without step 8, but has better if it is performed) → mature, hexacylated Lipid A
Step 1 is an unfavourable reaction, unlike step 2 which is irreversible. Step 2 is thus the central regulator step of the pathway. Between steps 7 and 8, WaaA adds 2 Kdo sugars to core of lipid IVa. This is not directly involved in Lipid A synthesis, but provides the first step in the synthesis of oligosaccharide. Further, after being flipped across the inner membrane, about ⅓ of Lipid A is modified by LpxT by using Und-PP to add a second phosphate group (9).
Core Oligosaccharide Synthesis
Core Oligosaccharide synthesis begins with the addition of 2 Kdo sugars to core of lipid IVa by WaaA between steps 7 and 8 of Lipid A synthesis. It continues after completion of Lipid A synthesis with the inner core followed by outer core synthesis, as follows (9):
Inner Core Synthesis
- WaaC then WaaF each extend the inner core by addition of 1 heptose residue.
- WaaP phosphorylates the heptose provided by WaaC
- WaaQ attaches another heptose to the second (WaaF) heptose
- WaaY phosphorylates heptose provided by WaaQ
Outer Core Synthesis
- WaaG adds heptose to second heptose (WaaF)
- WaaO and WaaB glycosyltransferases add a glucose & galactose respectively to heptose transferred by WaaG
- WaaJ/R adds another glucose [dependent on WaaB activity]
- WaaU adds final heptose residue to glucose from WaaJ/R step.
The final heptose acts as an O antigen acceptor one arrived in the outer leaflet of IM (9).
O-antigen Synthesis
Rather than being synthesized directly on the core-lipid A molecule, the O antigen is fully synthesized independently from the rest of the LPS molecule. The O antigen is first built stepwise on a lipid carrier molecule, undecaprenyl phosphate (Und-P), and is then transferred to the core oligosaccharide of the nascent LPS molecule in the periplasmic face of the IM. The routes taken to complete the O antigen vary amongst different organisms and even strains, but generally fall into three categories: the Wzy-dependent pathway, the ABC-dependent pathway, and the synthase-dependent pathway (9).
Wzy-Dependent Pathway
- Wzx flips Und-P-linked O units to the periplasmic face of the IM
- Wzy polymerizes O units on a single Und-P carrier molecule while Wzz controls polymer length
- WaaL ligates polymerized O-antigen to the core-lipid A acceptor at the outer leaflet of the IM
ABC-Dependent Pathway
The ABC-dependent pathway only requires a single initiation event per molecule of the polymerized O antigen. The entirety of the polymerization process is carried out in the cytoplasm (9).
- Glycosyltransferases polymerize the completed O antigen on a single Und-P carrier molecule in the inner leaflet of the IM using nucleotide-activated sugar donors
- ABC transporter flips the polymerized O-antigen-Und-PP molecule to the periplasmic face of the IM
- WaaL attaches the O-antigen portion to the core-lipid A molecule
Synthase-Dependent Pathway
The mechanistic details of the Synthase-dependent pathway are unclear, but the titular synthase of the pathway, WbbF, is presumed to simultaneously polymerize and translocate the O-antigen across the IM (9).
LPS Transport
At the inner membrane
After completion of LPS biosynthesis in the cytoplasm, it is transported from the inner leaflet of the inner membrane, across the periplasmic space, and to the outer leaflet of the outer membrane through a protein complex composed of seven LPS-transport (Lpt) proteins, collectively called LptB2FGCADE. LPS first enters the LptB2FG complex of the inner membrane, whose role is an ATP-binding cassette (ABC) transporter.(13) An ABC transporter is a family of proteins that is conserved in all domains of life and acts as a crucial component of the transport system as it functions to push LPS through the periplasmic bridge, a channel that connects the inner and outer membranes.(14) LptB exists as a dimer within the LptB2FG complex and serves as a cytoplasmic ATPase.(15) LptB has a nucleotide-binding site where it binds to ATP, and a catalytic region for ATP hydrolysis which produces the energy required to initiate LPS extraction from the inner leaflet of the inner membrane and towards the periplasmic bridge.(15) LptF and LptG of the LptB2FG complex each consists of six transmembrane segments and one periplasmic domain.(16) As integral transmembrane proteins, LptF and LptG are predominantly made of alpha helices to anchor into the inner membrane’s phospholipid bilayer. The C-terminus (cT) periplasmic domain of LptF interconnects with the N-terminus (nT) region of LptC via a β-jellyroll domain that fits inside of the β-jellyroll domain of LptC, and this structure serves as the beginning of the channel of the periplasmic bridge.(15)
Through the perisplasmic bridge
LPS exits the LptB2FG complex and enters the periplasmic bridge consisting of LptC, along with LptA and the nT domain of LptD.(15) The proteins of the periplasmic bridge are complexed with each other through their termini, specificaly with the cT domain of LptC interacting with the nT domain of LptA, and subsequently, the cT domain of LptA interacting with the nT domain of LptD.(15) The periplasmic bridge protein LptC has a large periplasmic domain of beta sheets and a single alpha helix domain that spans the inner membrane and is in complex with LptB2FG. The periplasmic domain of LptC consists of a β-jellyroll-like structure formed by 16 β-strands(16) with a hydrophobic core that is involved in LPS binding and transport to LptA. The β-jellyroll domain of LptC extends to stack on top of the same domain of LptF(15) to form the base of the periplasmic bridge and LptC is anchored to the inner membrane via a single transmembrane alpha helix that inserts between the LptG transmembrane domain (TM)1 and LptF TM5.(13) LPS continues to be transported up the periplasmic bridge to LptA and the nT domain of LptD, and eventually towards the outer membrane. Resembling LptC, the remaining periplasmic proteins LptA and the nT domain of LptD both form β-jellyroll structure and are composed of 15 antiparallel β-strands14 being slightly twisted, and 22 β-strands(16), respectively. A hydrophobic core is formed inside these β-jellyroll structures, stretching from LptC, through LptA, and the nT domain of LptD.(13) This continuous hydrophobic groove throughout the periplasmic bridge mediates the transport of LPS to the outer membrane by shielding the six fatty acyl chains in the lipid A portion of LPS from the aqueous environment of the periplasm.(15)
At the outer membrane
The outer membrane proteins LptD and LptE form a stable complex and function as the outer membrane translocon. This complex is the assembly component required for LPS assembly on the outer leaflet of the outer membrane.(17) Though the 22 β-strand structure in the nT domain of LptD resides in the periplasm, its cT domain forms a 26-stranded β-barrel structure in the outer membrane.(16) Additionally, the outer membrane lipoprotein LptE interacts with the β-barrel structure of LptD by residing within the cT β-barrel.(15) This “plug-and-barrel conformation” between LptD and LptE allows LPS to be transported across and inserted into the outer membrane.(15) Specifically, the O antigen and core oligosaccharides of LPS is translocated by the hydrophilic lumen of the LptD-E complex, and the hydrophobic lipid A portion of LPS is inserted through an intermembrane hole.(15) In order to have the outer membrane LptDE complex properly interact with LptA and function cohesively with the rest of the transport system, the correct folding and orientation of the nT and cT domains of LptD relative to the outer membrane is essential.(15) Specifically, the nT domain of the LptD must reside in the periplasm to interact with LptA and for the proper placement of LptDE in the outer membrane.(15) The proper folding of the nT and cT domains of LptD and its related function is achieved through oxidation reactions of LptD(16), forming two disulfide bonds. It has also been suggested that the involvement of LptE is essential for the correct oxidation and formation of the disulfide bonds in LptD.(15) When a functional LptDE complex interacts with LptA, it prevents the mislocalization of LPS as would be seen if LptA interacts with an improperly or incorrectly assembled LptDE complex.(15)
References
1. Doron S, Gorbach SL. Bacterial infections: overview. International Encyclopedia of Public Health [Internet]. 2008 [cited 2023 Feb 1];273–82. Available from: https://www.ncbi.nlm.nih.gov/pmc/articles/PMC7149789/
2. Kennedy AC. Bacterial diversity in agroecosystems. Agriculture, Ecosystems & Environment [Internet]. 1999 Jun 1 [cited 2023 Feb 1];74(1):65–76. Available from: https://www.sciencedirect.com/science/article/pii/S0167880999000304
3. Gram stain: what it is, purpose, procedure & results [Internet]. Cleveland Clinic. [cited 2023 Feb 1]. Available from: https://my.clevelandclinic.org/health/diagnostics/22612-gram-stain
4. Beveridge TJ. Structures of gram-negative cell walls and their derived membrane vesicles. J Bacteriol [Internet]. 1999 Aug 15 [cited 2023 Feb 1];181(16):4725–33. Available from: https://journals.asm.org/doi/10.1128/JB.181.16.4725-4733.1999
5. Sutcliffe IC. A phylum level perspective on bacterial cell envelope architecture. Trends in Microbiology [Internet]. 2010 Oct 1 [cited 2023 Feb 1];18(10):464–70. Available from: https://www.sciencedirect.com/science/article/pii/S0966842X10001058
6. Miller SI, Salama NR. The gram-negative bacterial periplasm: Size matters. PLoS Biol [Internet]. 2018 Jan 17 [cited 2023 Feb 1];16(1):e2004935. Available from: https://www.ncbi.nlm.nih.gov/pmc/articles/PMC5771553/
7. Silhavy TJ, Kahne D, Walker S. The bacterial cell envelope. Cold Spring Harb Perspect Biol [Internet]. 2010 May [cited 2023 Feb 1];2(5):a000414. Available from: https://www.ncbi.nlm.nih.gov/pmc/articles/PMC2857177/
8. Delcour AH. Outer membrane permeability and antibiotic resistance. Biochim Biophys Acta [Internet]. 2009 May [cited 2023 Feb 1];1794(5):808–16. Available from: https://www.ncbi.nlm.nih.gov/pmc/articles/PMC2696358/
9. Bertani B, Ruiz N. Function and biogenesis of lipopolysaccharides. Slauch JM, editor. EcoSal Plus [Internet]. 2018 Feb 8 [cited 2023 Feb 1];8(1):ecosalplus.ESP-0001-2018. Available from: https://journals.asm.org/doi/10.1128/ecosalplus.ESP-0001-2018
10. Montminy SW, Khan N, McGrath S, Walkowicz MJ, Sharp F, Conlon JE, et al. Virulence factors of Yersinia pestis are overcome by a strong lipopolysaccharide response. Nat Immunol. 2006 Oct;7(10):1066–73.
11. Murray GL, Attridge SR, Morona R. Altering the length of the lipopolysaccharide O antigen has an impact on the interaction of Salmonella enterica serovar Typhimurium with macrophages and complement. J Bacteriol. 2006 Apr;188(7):2735–9.
12. Iguchi A, Iyoda S, Seto K, Morita-Ishihara T, Scheutz F, Ohnishi M. Escherichia coli o-genotyping pcr: a comprehensive and practical platform for molecular o serogrouping. Bourbeau P, editor. J Clin Microbiol [Internet]. 2015 Aug [cited 2023 Feb 1];53(8):2427–32. Available from: https://journals.asm.org/doi/10.1128/JCM.00321-15
13.Owens TW, Taylor RJ, Pahil KS, Bertani BR, Ruiz N, Kruse AC, et al. Structural basis of unidirectional export of lipopolysaccharide to the cell surface. Nature. 2019 Mar;567(7749):550–3.
14. Okuda S, Sherman DJ, Silhavy TJ, Ruiz N, Kahne D. Lipopolysaccharide transport and assembly at the outer membrane: the PEZ model. Nat Rev Microbiol [Internet]. 2016 Jun [cited 2023 Feb 1];14(6):337–45. Available from: https://www.nature.com/articles/nrmicro.2016.25
15. Bishop RE. Ratcheting up lipopolysaccharide transport. Nature [Internet]. 2019 Mar [cited 2023 Feb 1];567(7749):471–2. Available from: https://www.nature.com/articles/d41586-019-00802-w
16. Dong H, Tang X, Zhang Z, Dong C. Structural insight into lipopolysaccharide transport from the Gram-negative bacterial inner membrane to the outer membrane. Biochimica et Biophysica Acta (BBA) - Molecular and Cell Biology of Lipids [Internet]. 2017 Nov 1 [cited 2023 Feb 1];1862(11):1461–7. Available from: https://www.sciencedirect.com/science/article/pii/S1388198117301506
17. Labonte AC, Kegerreis B, Geraci NS, Bachali P, Madamanchi S, Robl R, et al. Identification of alterations in macrophage activation associated with disease activity in systemic lupus erythematosus. Crispin JC, editor. PLOS ONE. 2018 Dec 18;13(12):e0208132.