This is an old revision of the document!
Table of Contents
Structural insights into outer membrane asymmetry maintenance in Gram-negative bacteria by MlaFEDB
This project is based on Tang et al.'s article, “Structural insights into outer membrane asymmetry maintenance in Gram-negative bacteria by MlaFEDB.” Please follow this External Link to access the article.
It is believed that the maintenance of lipid asymmetry (Mla) pathway is essential in outer membrane asymmetry by transporting phospholipids between the outer and inner membranes. However, the phospholipid trafficking direction in this pathway was not well-known. In their paper, Tang et al. (2020) investigated the Mla transport system in Gram-negative bacteria to determine its mechanism in outer membrane asymmetry maintenance.
Researchers
The main contributors to this investigation were Tang, Chang, Qiao, Zhang, and Dong.
Tang, Qiao, and Dong are from China's State Key Laboratory of Biotherapy and Cancer Center. They were involved in protein expression and purification, sample preparation, and ATPase and transport assays.
Chang and Zhang are from the Center of Cryo-EM Microscopy in China and thus led the cryo-EM aspect of the investigation.
Methods
Cryo-electron Microscopy
Cryo-electron microscopy (cryo-EM) is a powerful imaging technique used to determine and study the 3D structure of biological molecules and complexes. Unlike traditional electron microscopy imaging methods, which require the protein sample to be stained, fixed, and dehydrated, cryo-EM requires freezing the sample in a thin layer of ice fast enough that ice crystals cannot form. This allows proteins to remain in their natural soluble state. A beam of electrons is shot at the frozen sample, causing electron scattering to produce many 2D images of the protein from various angles. The unique shadows of the various angles are combined computationally to produce a 3D structure of the protein. One of the important advantages of using cryo-EM in determining protein structure is that it does not require crystallization of the protein sample. Obtaining optimal crystals for imaging using x-ray crystallography is one of the biggest hurdles to overcome, especially with large proteins and membrane complexes, due to their lack of stability, high flexibility, and partially hydrophobic surfaces. This is what makes cryo-EM the optimal imaging technique to determine the structure of a large membrane complex like MlaFEDB (Cheng, 2015).
Cryo-EM Grid Optimization
Grid optimization in cryo-em imaging is a limiting factor in determining protein structure. The article mentions some optimizations applied to the process, which include a blotting time of 3.5 seconds and a chamber environment with 95% humidity before the sample was flash-frozen in liquid ethane. These conditions are essential in obtaining the optimal thickness of the ice (Kampjut et al., 2021). The paper does not mention other potential optimizations that may improve the quality of the structures, such as using surfactants, which have been found to be very beneficial in obtaining high-quality structures of membrane proteins (Kampjut et al., 2021).
In vitro phospholipid transport assay
In vitro transport assays can be of 2 main types: membrane-based or cell-based. Membrane-based assays usually involve membranes that express the transporters, and are used to identify the substrates and inhibitors that are being effluxed (Volpe, 2015). Cell-based systems, on the other hand, are usually functional transporter assays that detect the transport of substances between membranes (Volpe, 2015).
In the case of an MlaFEDB assay, the MlaFEDB complex is an ABC transporter (ATB Binding Casette). Therefore, the assays take advantage of the conversion of ATP to ADP and inorganic phosphate, by the ATPase that they are associated with. Typical assays measure the amount of inorganic phosphate that is released.
FRET Assay
A Fluorescence Resonance Energy Transfer (FRET) assay takes advantage of the interaction between signalling proteins. The assay itself detect the oligomerization state and oligomerization degree of membrane proteins (“Fluorescence,” n.d.). It is dependent on the distance between the donor and acceptor molecules in a signalling cascade and is only effective if the distance between the donor and acceptor molecules is less than 10nm (“Fluorescence,” n.d.). Fluorescence energy is transferred from a donor molecule that is excited, to an acceptor molecule (“Fluorescence,” n.d.).
Cellular Sensitivity Assay
Cellular sensitivity assays are assays that are used to determine the sensitivity that cells have to certain substances. These are either used to determine the cytotoxicity of certain substances, or the rate of cell survival (Plumb, 1999). Cytotoxicity assays typically use trypan blue dye exclusion methods, or Cr release, and measure the functionality post-exposure (Plumb, 1999). Cell survival assays aim to evaluate whether the cells are able to survive and proliferate post-exposure, or whether they die (Plumb, 1999). The assay used in this paper was to measure the cell survival when mutated cells were exposed to chlorpromazine. The chlorpromazine was meant to serve as a membrane permeabilizer, to test whether certain residues in the complex were essential for the survival of the cell.
Results & Discussion
MlaFEDB mediates PL transport in vitro
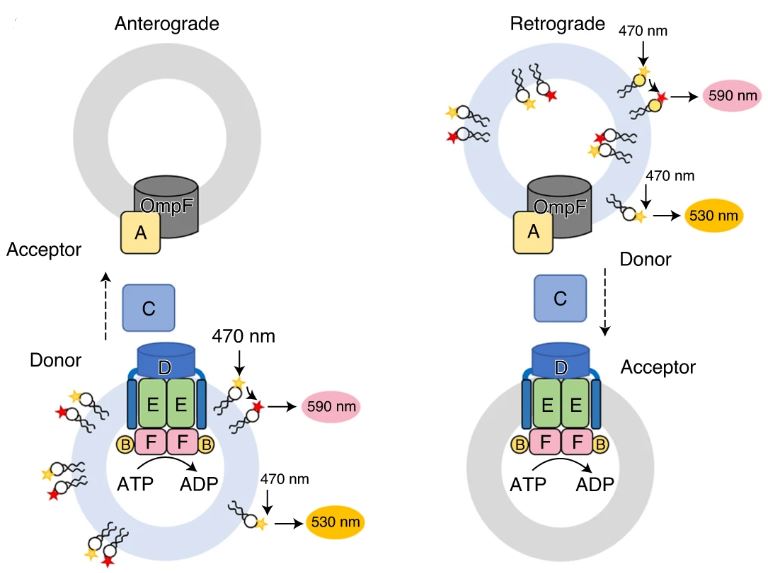
Figure 1: A diagram illustrating a fluorescence resonance energy transfer (FRET) transport assay of PL transport in anterograde (left) and retrograde (right) directions. A liposome is incorporated with MlaFEDB and MlaA–OmpF complexes, showing the movement of MlaC in the periplasm between the IM and OM. Adapted from Tang et al., 2021.
A K-12 E. coli strain was used to study the PL transport between the IM and the OM in vitro. The MlaFEDCB operon was used to express MlaFEDB which is the ABC (ABC-Binding Cassette) in the system. The complex had a higher ATPase activity in polar lipid liposomes from E. coli, than it did in micelles of n-dodecyl-β-d-maltoside (DDM). The complex was also seen to be inhibited by ADP or AMP-PNP.
An in vitro system was established to study the direction of movement of the PLs. The system consisted of the IM MlaFEDB complex, as well as OM porins such as the MlaA-OmpF complex. MlaC, the periplasmic transporter, was added to shuttle the PLs between the membranes. The IM and OM proteoliposomes were distinguished using E. coli polar lipids containing PE (phosphatidyl-ethanalomine), PG (phosphatidylglycerol), and CL (cardiolipin) or the non-bacterial POPC (phospholipid 1-palmitoyl-2-oleoyl-glycero-3 -phosphocholine), to help trace the PLs to the source of their origin. Since Mla proteins inherently contain PLs, the lipids were removed from MlaC, leading to the obtainment of its apo form before it was added to the assay. The lipids that were picked up for transport by MlaC were resolved using Thin Layer Chromatography (TLC) which greatly highlighted the transport of PE, PG, and POPC from the OM, and slightly highlighted the transport of PE and PG from the IM. It is important to note that MlaFEDB and MlaA-OmpF transport PLs to MlaC in their apo form since MlaC has a higher affinity for PLs than the complex does.
Fluorescent nitrobenzoxadiazole-labeled (NBD–PE) and rhodamine-labeled (Rhod–PE) Pes were used to measure the quantity of PLs transported. The labeled PEs was mixed with E. coli lipids for donors and unlabeled POPC for acceptor proteoliposomes. The proteins were then analyzed using Fluorescence Resonance Energy Transfer (FRET) assays. The results showed that the signal for NBD increased over time, meaning that there was a strong ATP-dependent transport of PLs taking place, from the OM to the IM. Since a retrograde transport direction meant that the PLs were being passed from the OM to IM, the results indicated that the ATPase activity of MlaFEDB strongly favored retrograde transport. However, it was seen that the rate of transport was influenced by the composition of the proteoliposomes from the IM. Empty proteoliposomes with a lack of MlaFEDB gave almost no signals while replacing the POPC with E. coli polar lipids greatly increased the rate of transport.
MlaA is believed to aid in the transport of PLs from the OM to MlaC, for subsequent transport to the IM. This is thought to be due to the H-loop that does not allow PLs to access the inner leaflet of the OM on their own. A lethal mutant of MlaA, MlaA*, (MlaA∆Asn41–Phe42) that has its Asn 41 and Phe 42 of the H-loop deleted, has been proposed to mediate PL flow from the inner to the outer leaflet of the OM. The FRET assays using the mutant, depicted in Fig. 1, showed reduced retrograde transport which was thought to be due to the blockage of MlaA.
Overall, it was concluded that transport of the PLs in the retrograde direction involved the entire MlaA-F system and that the PLs are particularly transported from the outer leaflet of the OM to the IM.
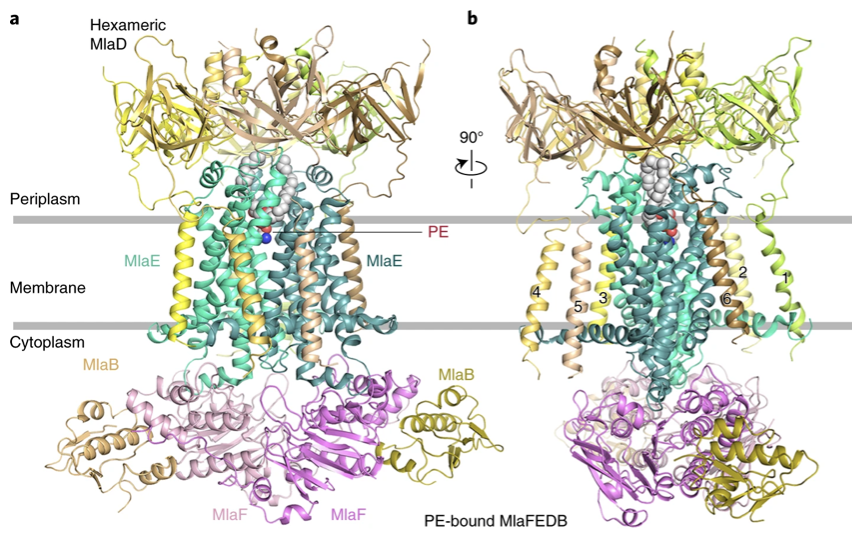
Figure 2a,b: Cartoon representation of the PE-bound MlaFEDB structure. Hexameric MlaD is shown in yellow, yellow-orange, wheat, sand, lemon, and pale yellow. The two MlaE molecules are shown in green-cyan and light teal. The two MlaF molecules are shown in violet and light pink. MlaB molecules are shown in olive and light orange. PE is shown as spheres. a. The TM domains of MlaD are shown and numbered. b, The PE-bound MlaFEDB complex rotated 90° along the y-axis relative to a.
The architecture of four MlaFEDB states
Tang et al. analyzed the MlaFEDB complex in four states, apo state, AMP-PNP bound, ADP bound states, and PL bound which provided resolutions of 3.6, 3.3, 3.3, and 4.1Å. The resolution is consistent throughout the molecule as shown in Fig. 2a,b, however, the quality of the structure can be improved with a resolution value below 3 Å. The researchers found that The hexameric structure of MlaD is on the periplasmic side and it resembles the shape of a lampshade. They also found a dimeric MlaF complex in the cytoplasm, at the base of the complex. One important thing they found was that MlaF interacts with MlaB, which we will discuss later on. Furthermore, they found the structures of MlaE. They found that each MlaE subunit consists of five transmembrane segments which lie on an N-terminal cytoplasmic alpha-helix elbow. This elbow helix is parallel to the plane of the inner membrane. Between transmembranes 3 and 4, there is a periplasmic loop that is found to interact with MlaF. Similarly, there is a cytoplasmic alpha-helix between transmembranes 2 and 3 which also interacts with MlaF. The structure also contains a cytoplasmic side, the MlaF forms a nucleotide-binding domain. The domain contains a helical domain and a Rec-A-like domain. They also found that the other three states were similar in conformation and structure.
MlaFEDB transports PLs toward the IM in a head-down orientation
TM1, TM2 and TM5 of the MlaE dimer creates a V-shaped cavity in the MlaFEDB complex. The cavity extends into the periplasm and therefore contains a channel within the hexameric disc of MlaD. The cavity mainly consists of hydrophobic residues that face inwards, with a few polar residues present towards the middle. The residues involved in the cavity are Tyr 81, Met 89, Val 77, Leu 90, Leu 93, Arg 97, Glu 98, Leu 99, Val 102 and Val 103.
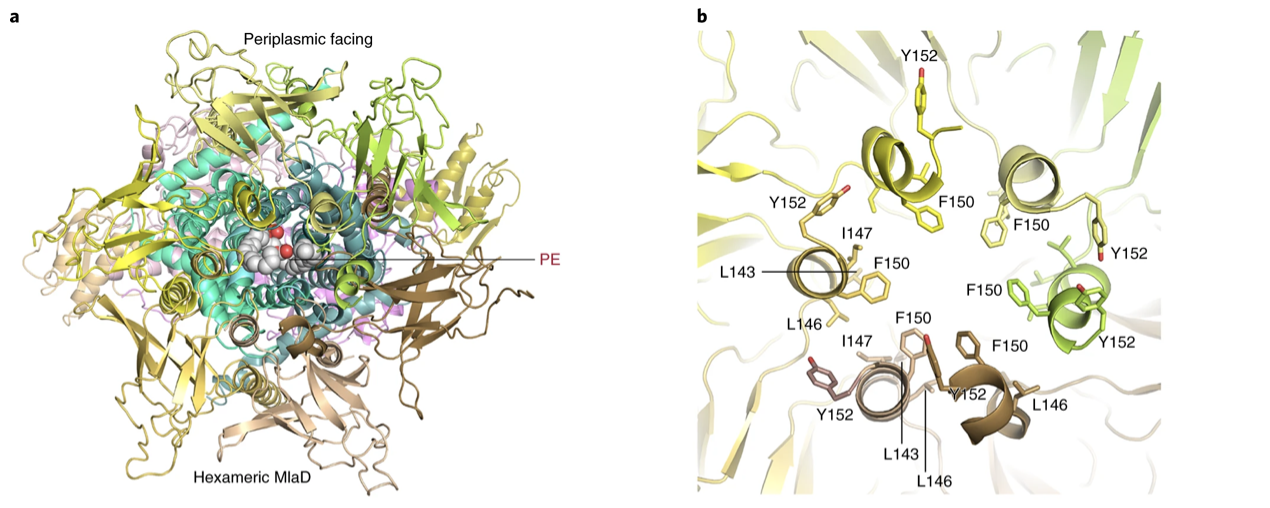
Figure 3a,b: Periplasmic view of MlaFEDB showing bound PE. b, The periplasmic central channel of hexameric MlaD showing the hydrophobic residues Leu 143, Ile 147, and Phe 150 facing inside the channel
When MlaFEDB is bound to a PL, PEs are mainly found within the cavity with the polar phosphate group facing downwards. Two acyl chains are seen to interact with the hydrophobic residues (Gln73, Arg97, Leu93, Leu90, Val 77, Met 89 and Tyr 81) in the upper cavity. To determine which of the substrate binding residues are vital to the transport of PL, various single mutations were made and assayed using an in vitro PL transport assay as well as an in vivo cellular sensitivity assay. It was found that Y81E and V77E mutants that had their acyl chain-interacting residues altered, and E98R that had a substitution in a polar head-interacting residue led to the diminishing to the retrograde transport of PLs, along with increased cell death due to the chlorpromazine used in the cellular sensitivity assay. The E98R substitution was seen to increase anterograde transport from MlaFEDB to MlaC. This opened up the possibility of MlaFEDB also having the ability to adapt to PL in another orientation.
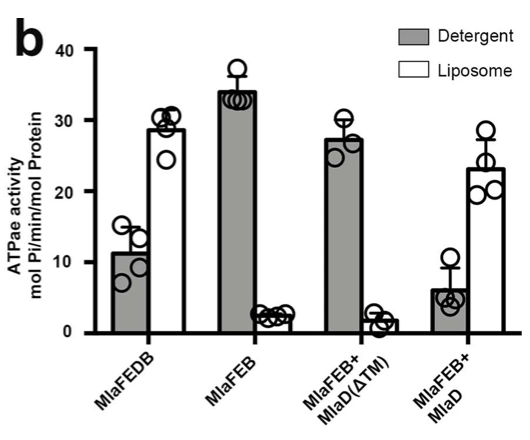
Extended Figure 1: The relative ATPase activities of wild-type, MlaFEB, and in vitro formed MlaFEDB were measured in detergent and liposomes. Soluble MlaD(ΔTM) was added into MlaFEB-constructed systems and its effect on ATPase activity was also analyzed.
MlaD regulates MlaFEB ATPase and transport activity
The C-terminal of the MlaD subunit interacts with the TMDs of the overall complex in the periplasmic space via loops 1 and 2 of MlaE (Figure 2a,b). Each MlaE protomer interacts with the TM segments from three adjacent MlaD subunits. The interaction occurs at conserved residues Gly10 and Gly21 of the elbow helix and the surface residues of TM1 and TM3. Each MlaD contains a TM segment at its N-terminus. In all forms of the MlaFEDB structures that were visualized via cryo-EM (apo, PL-bound and AMP-PNP-bound), six TM segments from MlaD are observed (Fig. 2a,b).
It was also found that because MlaFEB showed higher ATPase activity than MlaFEDB in detergent micelles, it was determined that MlaD serves a regulatory role in ATPase activity.
When the MlaD coding region that corresponded to the TM helix of MlaD was deleted, MlaD could no longer be purified complexed to MlaFEB which suggests that its regulatory role is for stable complex formation (Extended Fig. 1).
Additionally, residues Leu 143–Gly 153 of each MlaD subunit form a short helix in the periplasmic domain. These residues associate with each other to create a channel along the middle of the MlaD hexamer (Fig. 3a,b). When the amino acids, Leu 143–Gly 153 of MlaD were mutated, MlaD lost its hexameric form and retrograde PL transport activity was no longer possible.
It was also found that F150 was not essential for PL transport as when it was mutated to F150E, PL transport slowed but cell survival was still possible.
Overall, the results confirm that MlaD is a mammalian cell entry (MCE) protein that forms hydrophobic channels and transports hydrophobic molecules. The results also show that the MlaD subunit regulates MlaFEB ATPase and transport activity.
MlaB stabilizes and regulates the MlaFED transporter
The structure of MlaB is comprised of three α-helices and four β-strands. The cryo-EM structures revealed that the interface area of interaction between MlaB and MlaF was 768.4 Å^2 and included crucial hydrophobic and polar interactions. To examine the importance of this interaction single-residue MlaB mutants with substitutions of a polar residue T52A (Threonine to alanine (Polar to non-polar) and hydrophobic residues W29E (tryptophan to glutamic acid (non-polar to charged) and Y88E (Tyrosine to glutamic acid (polar to charged) were generated in the MlaFEDB complex. During the purification process, the W29E and Y88E MlaFEDB mutants were not purified, suggesting that the hydrophobic interaction facilitated by these key residues is essential for the stability of the MlaFEDB complex. The T52A mutant was purified, and ATPase activity and transport assays were performed. It was revealed that the substitution mutation diminished the ATPase activity of the protein complex as well as its ability to transport PLs (Extended figure 2a,b). The models show that MlaB binds to the α-helical regions of MlaF, which then interact with the coupling helices of MlaE, suggesting that MlaB plays an important role in stabilizing the entire rest of the MlaFEDB complex.
Binding of ADP and AMP-PNP in MlaF
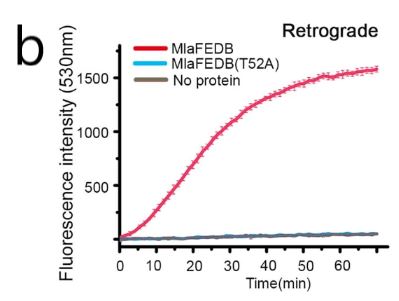
Extended figure 2b: FRET scan depicting the retrograde transport of PLs when using the wild-type MlaFEDB (red) and the mutant MlaFEDB(T52A) (blue) in conditions containing ATP AND MgCl2.
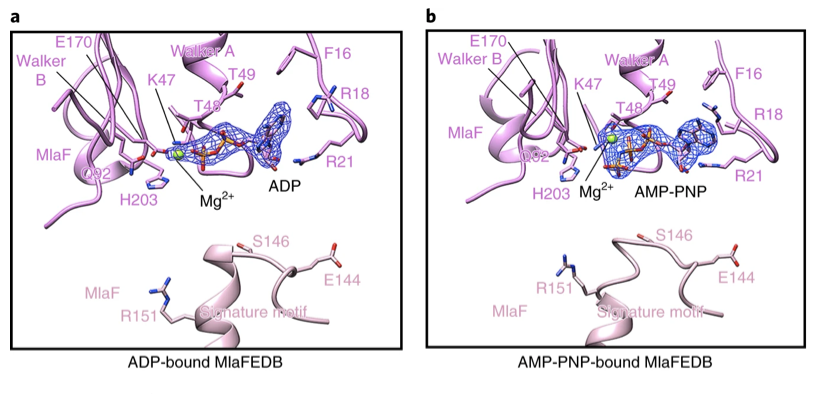
Figure 4a,b:Residues binding ADP (a) or AMP-PNP (b) and Mg2+ ions in the catalytic site of one MlaF subunit and the signature motif of the other MlaF subunit containing conserved residues Glu 144, Ser 146 and Arg 151.
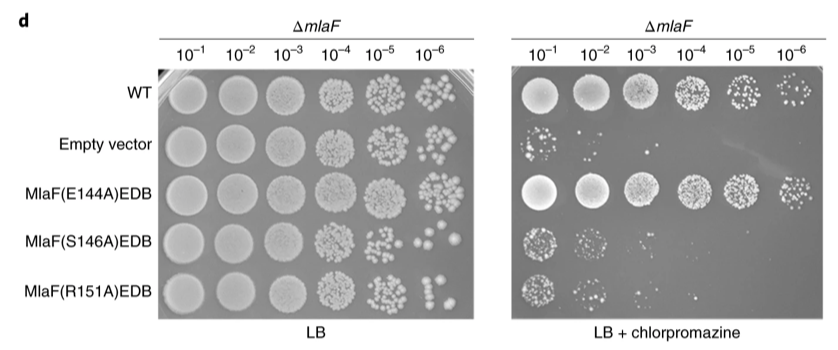
Extended Figure 3: Cell growth assays of cells expressing MlaF mutated at signature residues in the context of an MlaF deletion strain. The assays were performed on LB plates without (left) or with (right) 120 μg ml−1 chlorpromazine.
MlaF subunits use Mg2+ ion cofactors and ATP/ADP interact with residues in the active site of the nucleotide-binding domain (NBDs) (Figure 4a,b). The adenine ring of the nucleotide interacts with Phe 16 and Arg 18 of MlaF, and the ribose interacts with Arg 21 and Ile 23. Single residue substitutions of charged amino acids to Ala, impair ATPase activity and in vitro transport activity of the overall MlaFEDB complex.
The C-terminal of MlaF is longer than its homolog, LptB, and the extra residues of MlaF allow it to interact with MlaB and the other MlaF subunit. It was determined that even though the C-terminal is not involved in the ATPase or transport function of the overall complex, it plays a key role in stabilizing the complex as it could not be purified when the C-terminal was deleted. It was also found that S146A and R151A substitutions truncated PL transport and cell survival when chlorpromazine was introduced (Extended figure 3). Overall, the authors found that ATP hydrolysis may be required for Mla complex conformational change to occur.
Limitations and Future Directions
In terms of general critiques, Tang et al. lacked in the organization of the paper. The results are not communicated effectively to the readers, which is especially present with the figures and figure captions. The figures are scattered across the paper, making it difficult to connect the results mentioned to supplementary data. For example, the caption for Figure 1 is on a separate page from the figure itself. This makes the paper appear messy and hinders the overall organization, resulting in an overall incoherent read. In addition, the general information provided in the paper does not flow well, and sufficient background information in order to understand the results was lacking.
In terms of results, the paper showed the ATP-dependent retrograde transport of phospholipids from MlaA-OmpF to MlaFEDB and the anterograde diffusive flow of phospholipids from MlaFEDB to MlaC. However, it is uncertain if the anterograde diffusion between MlaFEDB and MlaC that was observed in their in-vitro assay is actually physiologically relevant. Upon observation of cells expressing the MlaA* mutant, for example, high-flux anterograde flow between the inner and outer membranes is still seen. This occurs through YhdP–another MlaA-associated protein, suggesting redundancy. To identify genes responsible for affecting phospholipid transport, researchers can screen for genetic interactions with MlaA* and once associated proteins are found, observe what happens when those proteins are deleted to understand how this pathway is modulated. A paper by Ruiz et al., who studied the redundant proteins YhdP, TamB, and YdbH, found that they are actually essential to maintain outer membrane lipid homeostasis (Ruiz et al., 2021).
Although Tang et al. concluded that the C-terminus of the MlaFEDB complex did not play a functional role in the complex, they were unable to purify the MlaFEDB complex with the C-terminal tail deleted. They suggested that the C-terminal tail plays a role in stabilizing the complex, but did not elaborate any further. Further studies can be done to examine the structural differences seen upon cleavage of the C-terminus. For example, a study by Ekiert et al. used X-ray Crystallography and electron microscopy to look into this. They found that while MlaD usually forms a hexameric ring that facilitates its hydrophobic core, cutting out the C-terminus results in heptameric rings instead (Ekiert et al., 2017). This suggested that the C-terminus played a role in determining the oligomeric state and the packing of the MCE subunits themselves (Ekiert et al., 2017).
The information and results provided by Tang et al.’s paper can also be utilized in future studies to explore pathways in different types of bacteria such as gram-positive bacteria. For example, future studies can look into the functions of MlaFEDB in gram-positive bacteria. An article by Zhou et al. reported the Cryo-EM structure of the MlaFEDB transport system in P.areuginosa. Furthermore, they studied MlaFEBD functions in this gram-positive bacteria. From these studies, future studies can try to grasp and understand in vivo function and apply it to therapeutic research (Zhou et al., 2021).
References
Cheng, Y. (2015). Single-particle cryo-EM at crystallographic resolution. Cell, 161(3), 450–457. https://doi.org/10.1016/j.cell.2015.03.049
Ekiert, D. C., Bhabha, G., Isom, G. L., Greenan, G., Ovchinnikov, S., Henderson, I. R., Cox, J. S., & Vale, R. D. (2017). Architectures of lipid transport systems for the bacterial outer membrane. Cell, 169(2). https://doi.org/10.1016/j.cell.2017.03.019
Fluorescence Resonance Energy Transfer (FRET) Assay. Fluorescence resonance energy transfer (FRET) assay. (n.d.). Retrieved March 2, 2023, from https://www.creative-biostructure.com/fluorescence-resonance-energy-transfer-fret-assay-306.htm
Kampjut, D., Steiner, J., & Sazanov, L. A. (2021). Cryo-EM grid optimization for membrane proteins. IScience, 24(3), 102139. https://doi.org/10.1016/j.isci.2021.102139
Plumb, J. A. (1999, January 1). Cell sensitivity assays. SpringerLink. Retrieved March 2, 2023, from https://link.springer.com/protocol/10.1385/1-59259-687-8:17
Ruiz, Natividad, Rebecca M. Davis, and Sujeet Kumar. 2021. “YhdP, TamB, and YdbH Are Redundant but Essential for Growth and Lipid Homeostasis of the Gram-Negative Outer Membrane” edited by S. Gottesman. MBio 12(6):e02714-21. doi: 10.1128/mBio.02714-21.
Tang, X., Chang, S., Qiao, W., Luo, Q., Chen, Y., Jia, Z., Coleman, J., Zhang, K., Wang, T., Zhang, Z., Zhang, C., Zhu, X., Wei, X., Dong, C., Zhang, X., & Dong, H. (2020). Structural insights into outer membrane asymmetry maintenance in gram-negative bacteria by MlaFEDB. Nature Structural & Molecular Biology, 28(1), 81–91. https://doi.org/10.1038/s41594-020-00532-y
Volpe, D. A. (2015, October 29). Transporter assays as useful in vitro tools in drug discovery and development. Taylor & Francis. Retrieved March 2, 2023, from https://www.tandfonline.com/doi/full/10.1517/17460441.2016.1101064
Zhou, Changping, Huigang Shi, Manfeng Zhang, Lijun Zhou, Le Xiao, Shasha Feng, Wonpil Im, Min Zhou, Xinzheng Zhang, and Yihua Huang. 2021. “Structural Insight into Phospholipid Transport by the MlaFEBD Complex from P. Aeruginosa.” Journal of Molecular Biology 433(13):166986. doi: 10.1016/j.jmb.2021.166986.