Table of Contents
Gram-Negative Bacteria: Maintenance of Outer Membrane Asymmetry
This project is based on Tang et al.'s article, “Structural insights into outer membrane asymmetry maintenance in Gram-negative bacteria by MlaFEDB.” Please follow this External Link to access the article.
It is believed that the maintenance of lipid asymmetry (Mla) pathway is essential in outer membrane asymmetry by transporting phospholipids between the outer and inner membranes. However, the phospholipid trafficking direction in this pathway was not well-known. In their paper, Tang et al. (2020) investigated the Mla transport system in Gram-negative bacteria to determine its mechanism in outer membrane asymmetry maintenance.
Gram-Negative Bacterial Cell Envelope
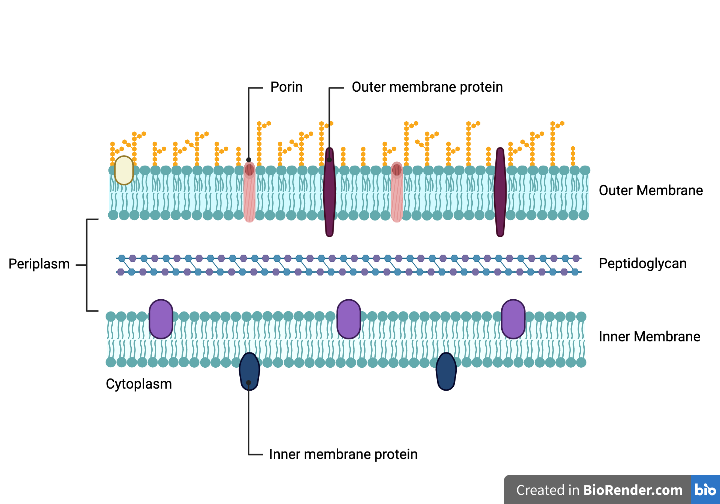
Figure 1: Gram-negative bacterial cell envelope. The three layers include the outer membrane, peptidoglycan, and the inner membrane. Each play an important role in the cell's survival. Adapted from Libretexts, 2022.
Through evolution, bacteria have developed a complex cell envelope that supports their survival. The envelope of Gram-negative bacteria serves as protection and allows selective passage of nutrients from the outside environment and waste products from inside the cell (Silhavy, 2010). There are three layers in the cell envelope: the outer membrane (OM), peptidoglycan, and the inner membrane (IM) (Silhavy, 2010). Each layer plays an important role in transportation and protection (Silhavy, 2010).
The Outer Membrane
The (OM) is the distinguishing feature of Gram-negative bacteria compared to Gram-positive bacteria, which lacks an outer membrane (Silhavy, 2010). Its only known function is to serve as a protective barrier. This membrane is highly organized and is composed of an outer and inner leaflet, resulting in asymmetry (Silhavy, 2010). A leaflet is a single layer of lipids, and a stable membrane requires two opposing leaflets (Silhavy, 2010). The outer leaflet of the OM is composed of lipopolysaccharides (LPS) with a glycolipid (Silhavy, 2010). Lipid A, the glycolipid, attaches LPS to the outer membrane (Silhavy, 2010). The OM has a larger overall density than the IM because of the glycoproteins in the outer leaflet (Silhavy, 2010). Proteins in the OM are categorized into lipoproteins and β-barrel proteins, such as porins (Silhavy, 2010). Porins span the outer membrane through both leaflets, facilitating the passive diffusion of small hydrophilic molecules (Silhavy, 2010). The inner leaflet of the OM is composed of phospholipids (Hobot, 2015). Lipoproteins are also found in the inner leaflet and contribute to the attachment between the OM and peptidoglycan (Hobot, 2015).
The asymmetric nature of the OM is essential in the selective passage of molecules (Silhavy, 2010). LPS forms a continuum, preventing hydrophobic molecules from entering (Silhavy, 2010). Porins in the OM compound this restriction by limiting the diffusion of large hydrophobic molecules (Silhavy, 2010). The internal hydrophilic channel of porins allows the passage of smaller macromolecules, such as glucose, into the cell (Hobot, 2015). Therefore, the OM is incredibly selective in terms of passage and protects the bacteria from cytotoxic substances. The maintenance of asymmetry is vital for bacterial survival and is of great interest for antimicrobial drug development. Gram-negative bacteria maintain this asymmetry via specific pathways that transport essential molecules to the OM (Silhavy, 2010).
Peptidoglycan
In the periplasm, the peptidoglycan wall sits between the OM and IM. Due to its rigidity, the wall provides the cell's shape (Silhavy, 2010). The peptidoglycan wall is made of repeating units of N-acetylglucosamine and N-acetylmuramic acid (Silhavy, 2010). Pentapeptide side chains cross-link these units (Silhavy, 2010). Murein lipoprotein (Lpp) acts as an adhesive and connects the OM to the peptidoglycan wall. Lpp is embedded in the OM by connecting lipids, which are attached to the amino terminus of the protein (Silhavy, 2010). Additionally, OM proteins, such as OmpA, bind peptidoglycan noncovalently (Silhavy, 2010).
Periplasm
The periplasm is the aqueous space between the OM and IM and is densely packed with proteins (Silhavy, 2010). Compartmentalization allows Gram-negative bacteria to isolate threats such as harmful enzymes (Silhavy, 2010). Other proteins in the periplasm include periplasmic binding proteins, which help facilitate sugar and amino acid transport and chemotaxis, and chaperone-like molecules that support membrane biosynthesis (Silhavy, 2010).
The Inner Membrane
The IM is a phospholipid bilayer with symmetric properties (Silhavy, 2010). This membrane primarily comprises phosphatidyl ethanolamine and phosphatidyl glycerol, with small amounts of phosphatidyl serine and cardiolipin (Silhavy, 2010). The IM proteins are involved in energy production, lipid biosynthesis, and transport (Silhavy, 2010). Crossing through the cell envelope requires passage through the OM porins, periplasm, and IM (Silhavy, 2010). The IM transport proteins are different than the OM ones. For example, the IM uses ABC (ATP-binding cassette) proteins, transporters that are driven by ATP transport systems (Silhavy, 2010).
The Mla Pathway
The multicomponent maintenance of lipid asymmetry (Mla) pathway, is an important system used to maintain the lipid composition of bacterial cell walls (Mann et al., 2021). This process is done through trafficking phospholipids between leaflets and this supposedly requires a significant amount of energy, whereby the hydrolysis of ATP was commonly thought to be coupled with this process (Hughes et al., 2019; Locher, 2009; Mann et al., 2021).
Peripheral Structure and Function of Mla Pathway
The Mla pathway is a six-protein system (Hughes et al., 2019). There is much research in elucidating the structure and function of the Mla pathway; the peripheral components have been well understood but not the core system (Mann et al., 2021). The outer membrane contains the MlaA-OmpC-OmpF (major porins) complex, and the periplasmic space contains the soluble MlaC which acts as the shuttle between the leaflets (Hughes et al., 2019; Mann et al., 2021). It has been seen, in the Apo conformation of MlaC, that there is a pivoting β-sheet mechanism which opens and closes the phospholipid binding region, revealing the hydrophobic pocket (Hughes et al., 2019). Although the research on transport directionality is ongoing, the structure of the MlaFEDB system located within the inner membrane has been identified (Ekiert et al., 2022; Mann et al., 2021).
ABC Transport Superfamily
The MlaFEDB complex is considered the core of the system where ATP conversion to lateral transport occurs and this structure is highly conserved within the bacterial species (Ekiert et al., 2022; Mann et al., 2021). This complex is part of the ATP-binding cassette (ABC) transporter superfamily (Ekiert et al., 2022; Locher, 2009; Mann et al., 2021). ABC transporters convert energy from ATP hydrolysis into transbilayer movement of substrates; this ATP conversion is catalyzed by a pair of cytoplasmic ABCs, also known as nucleotide-binding domains (Locher, 2009). Like other ABC transporters, it consists of the transmembrane proteins that facilitate the translocation of the substrate, membrane-associated ATPases, and integral membrane proteins that import and export substrates through the complex (Locher, 2009).
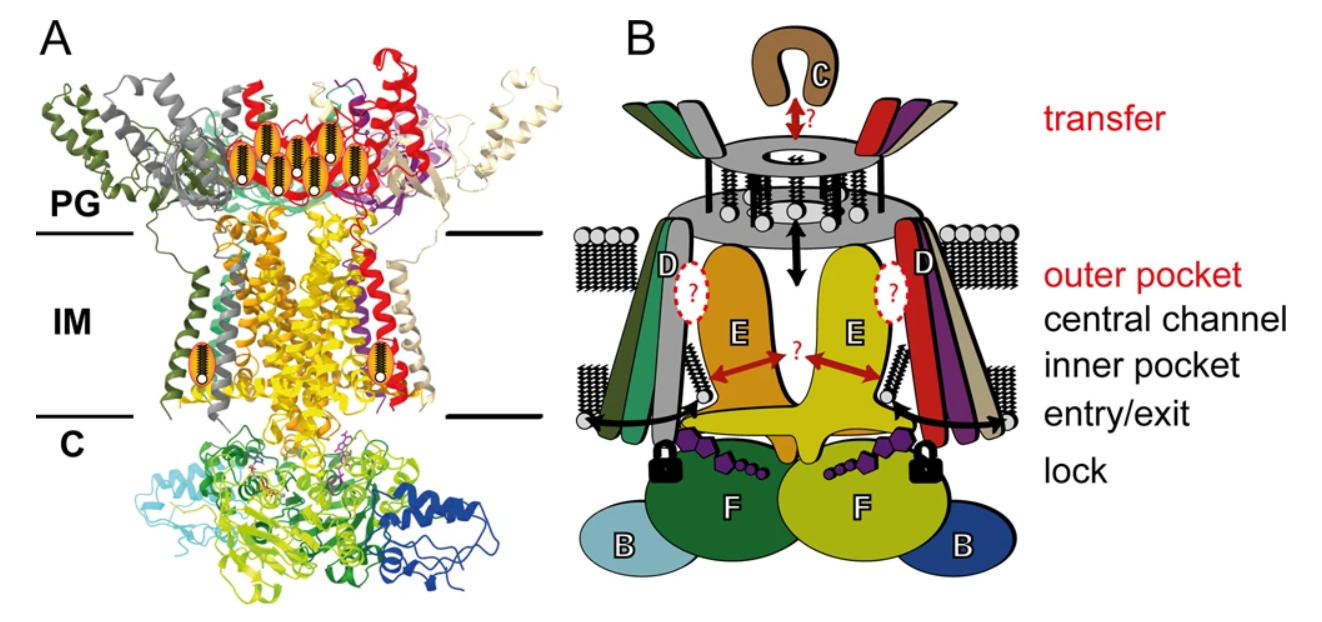
Figure 2: Molecular model of the MlaFEDB complex. (A) Ribbon structure of complex and (B) the cartoon representation of the MlaFEDB complex with the MlaC protein. Displayed are the 12 subunits that make up the MlaFEDB complex with the predicted regions where phospholipid binding occurs. The entry/exit point through the hexagonal basket structure, MlaD, is explicitly shown (black arrow) without certainty in the directionality. The structure is also observed to have a certain degree of structural movement. Adapted from Mann et al., 2021.
Structure of the MlaFEDB Complex
The MlaFEBD complex contains 12 subunits (Ekiert et al., 2022). On the cytosolic side, there is the transmembrane subunit, a homodimer MlaE, which is anchored into the MlaF protein of the paired ATPases, MlaF, via a coupling helix (Ekiert et al., 2022; Mann et al., 2021). It has been determined that the helices of the paired MlaE run parallel to the membrane and do not cross it (Mann et al., 2021). MlaB is a small sulfate transporter composed of anti-sigma factor antagonist domains, and it binds to each of the two copies of MlaF away from the nucleotide-binding site (Ekiert et al., 2022; Mann et al., 2021). However, it has been demonstrated that about 50% of the particles would bind MlaF to both MlaB, whereas, the other 50% of the time one MlaB binding site would be unoccupied (Mann et al., 2021). Sitting atop the periplasmic end of the transporter is a symmetrical hexagonal basket structure, MlaD—a ring-shaped homohexamer formed by six identical MCE domain proteins (Ekiert et al., 2022; Mann et al., 2021). The N-terminal spans the entirety of the IM, and it is in this space where MlaD is anchored by a single transmembrane (TM) helix from each MCE domain (Ekiert et al., 2022; Mann et al., 2021). Three TM helices of MlaD wrap around each of the two MlaE molecules and interact in an asymmetrical manner. It has been identified that of the six MlaD TM helices, TM1 does not traverse the membrane; instead, it is embedded in IM (Mann et al., 2021).
MlaFEDB Binding Sites
As stated previously, the MlaD structure forms a hexagonal basket structure, where hydrophobic residues narrow down the structure to the center creating a pore-like component (Mann et al., 2021). This center region has paired lipid binding sites (Mann et al., 2021). The N-terminal and C-terminal helices of the MlaE as well as the N-terminus of the MlaD form each of the binding pockets (Mann et al., 2021). Although the question of whether substrates are exported or imported is controversial, this would be the location of where the process takes place (Hughes et al., 2019).
PL Transport
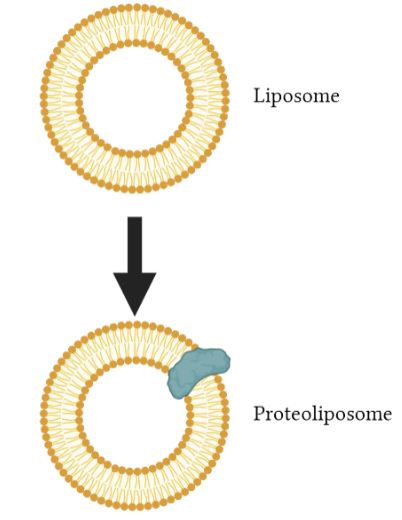
Figure 3: An illustration of a liposome and a proteoliposome with a membrane protein embedded. Image created using BioRender.
Liposomes
Liposomes are systems that imitate lipid membranes. They are made of a lipid bilayer and are small vesicles in a spherical shape, as shown in the figure presented. These liposomes are produced for encapsulating and transporting medicines, making a big contribution to the field of drug delivery. An example of a use of liposomes is in transporting medicines such as antibiotics or enzymes into cells for certain therapeutic effects (Ciancaglini et al., 2012).
Proteoliposomes
Proteoliposomes on the other hand, consist of liposomes where a protein is added or inserted into the lipid bilayer. For example, transporters can be added into these artificial liposomes to research how proteins behave and what they are responsible for transporting. They are used as a method to study membrane transporters in an isolated environment (Ciancaglini et al., 2012). In relation to the article by Tang et al., the entire Mla system was inserted into liposomes to make proteoliposomes. A proteoliposome-based transport system was then utilized to monitor the transport direction of phospholipids. It was used to determine the direction of transport, whether it is anterograde or retrograde transport of PLs.
Direction of PL Transport
The direction of phospholipid transport by the Mla pathway between the inner and outer membranes of gram-negative bacteria has been an issue of debate due to conflicting evidence from various studies over the years. The retrograde hypothesis suggests that PLs are transported by MlaC across the periplasm from the OM to the IM, while the anterograde flow is from the IM to the OM.
Evidence in Support of Retrograde PL Transport
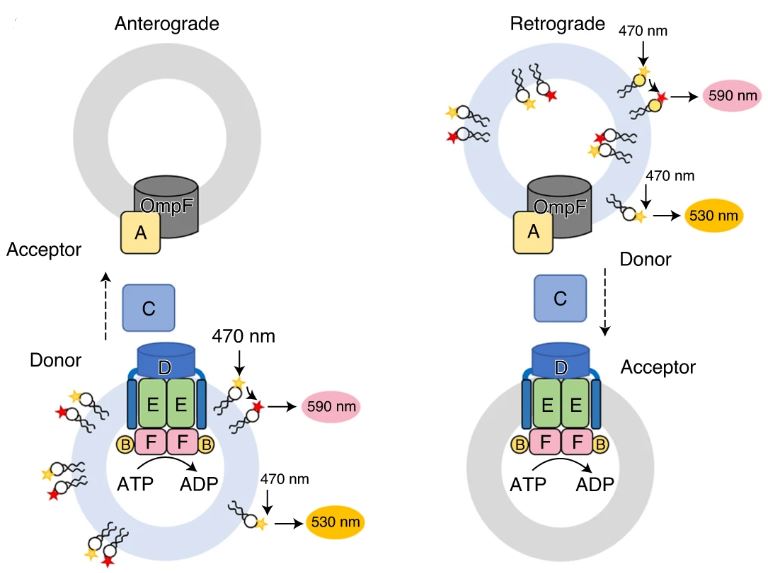
Figure 4: A diagram illustrating a fluorescence resonance energy transfer (FRET) transport assay of PL transport in anterograde (left) and retrograde (right) directions. A liposome is incorporated with MlaFEDB and MlaA–OmpF complexes, showing the movement of MlaC in the periplasm between the IM and OM. Adapted from Tang et al., 2021.
A study by Juliana C. Malinverni and Thomas J. Silhavy conducted using Escherichia coli found that when one of the components of the Mla pathway is removed, the outer leaflet of the OM accumulated phospholipids, suggesting that due to the malfunctioned Mla pathway, retrograde transport of PL from the OM to the IM is halted. Another study by Abellón-Ruiz, J. et al. in support retrograde PL transport hypothesis found that the MlaA protein located in the inner leaflet of the outer membrane selectively removes PLs from the outer leaflet and mediates PL transport via MlaC. Furthermore, they found that a lethal mutation in the MlaA gene interrupted the flow of PLs between the inner and outer leaflets of the OM (Abellón-Ruiz et al., 2017).
Evidence in Support of Anterograde PL Transport
An investigation by Kamischke, C. et al. suggests the contrary. They analyzed the Mla pathway in Acinetobacter baumannii, screened many transposon mutants, and picked out specific mutants showing defects in OM development. An example was the MlaC gene deletion, which resulted in the accumulation of PLs in the inner membrane, suggesting that MlaC trafficked PLs in an anterograde flow. Additional work by Hughes et al. found that MlaD embedded in the IM passes PLs onto MlaC in the periplasm. What is common between either hypothesis is that MlaC functions in the periplasm in trafficking PLs between MlaD and MlaA (Kamischke et al., 2019).
The Position of Tang et al.
In the main article, Tang et al. attempt to tackle this mystery using a proteoliposome-based transport system that contains the Mla components (MlaFEDB, MlaC, and MlaA) as a tool to investigate the direction of PL transport. The findings of Tang et al. using transport and other functional assays strongly suggest that the ATP-dependent transport of PLs occurs in retrograde at a much greater rate than the diffusion rate (ATP-independent).
The Importance of Determining Direction of PL Transport
Determining the direction of PL transport by the Mla pathway will help in understanding the intricacies of the system and the precise mechanism by which each component contributes to the ultimate goal of maintaining the lipid asymmetry of the OM. The more we know about each component, the greater potential they have in serving as a target for effective antimicrobial therapeutics.
Antibiotics
Antibiotics & Resistance
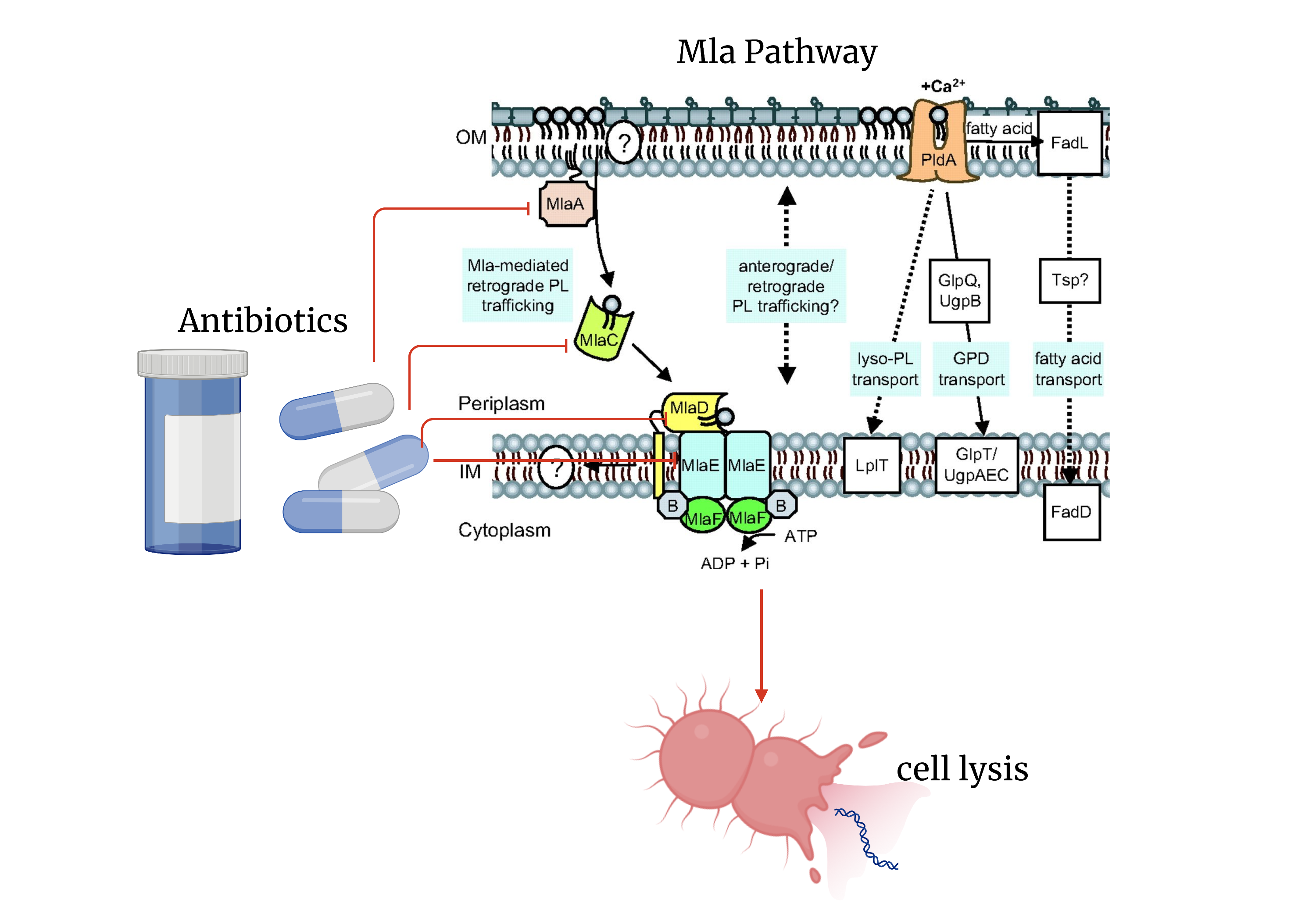
Figure 5: Possible antibiotic targets and the effects of targeting the Mla pathway to combat gram-negative bacterial infection. Including a figure from (Malinverni and Silhave, 2009). Image created in Biorender.com.
Antibiotics are drugs that are used to fight bacterial infections. They were first discovered by Alexander Fleming in 1928 (Hutchinson et al., 2019). While their discovery changed modern medicine, they also became the most commonly prescribed medication (Cleveland Clinic, 2016). This resulted in the overuse of antibiotics which has resulted in the emergence of antibiotic resistance. The overuse of antibiotics allows for the selection of resistant bacteria with adaptations that can render antibiotics ineffective (Walsh & Wencewicz, 2020).
How do Antibiotics Work?
Antibiotics work by blocking or inhibiting vital processes that bacteria require for survival. They either kill the bacteria – bactericidal – or stop the growth/spread of the bacteria – bacteriostatic. Both options allow the human immune system to then clear the infection from the body (NPS MedicineWise, 2017).
Antibiotics & Gram-negative Bacteria
The focus of the paper is on the Mla transport protein which is relevant for gram-negative bacteria. Some common examples of gram-negative bacteria include Acinetobacter spp., Escherichia coli, Salmonella spp., Helicobacter pylori, etc. These bacteria have been targeted by some antibiotics like Aminoglycosides, Polymyxins, Tetracyclines, Fosfomycin, etc., but resistance has developed to some of these antibiotics (Chopra et al., 2001) and so, the work by Tang et al. (2021) presents information about the Mla pathway which can be a potential target for antibiotic development.
Mla Pathway and Antibiotics
Outer membrane lipid asymmetry allows gram-negative bacteria to resist detergents and limits antibiotic access to the bacterial IM (Bishop, 2021). The selective nature of the outer membrane is facilitated by the Mla protein system (Tang et al., 2021). Targeting the Mla protein subunits could make way for combination antibiotic therapies that would then allow for the elimination of gram-negative bacteria. The steps currently being taken in understanding whether the Mla system functions in an anterograde or retrograde is a step in the right direction in determining targets for antibiotics.
References
Abellón-Ruiz, J., Kaptan, S. S., Baslé, A., Claudi, B., Bumann, D., Kleinekathöfer, U., & van den Berg, B. (2017). Structural basis for maintenance of bacterial outer membrane lipid asymmetry. Nature Microbiology, 2(12), 1616–1623. https://doi.org/10.1038/s41564-017-0046-x
Antibiotics, explained. NPS MedicineWise. (2017). Retrieved January 31, 2023, from https://www.nps.org.au/consumers/antibiotics-explained
Antibiotics: Side effects, what is it & usage. Cleveland Clinic. (2016). Retrieved February 2, 2023, from https://my.clevelandclinic.org/health/drugs/16386-antibiotics
Bishop, R. E. (2021). Phospholipid transporter shifts into reverse. Nature Structural & Molecular Biology, 28(1), 8-10. Canada, P. H. A. of. (2021). Government of Canada. Canada.ca. Retrieved February 2, 2023, from https://www.canada.ca/en/public-health/services/antibiotic-antimicrobial-resistance/about-antibiotic-resistance.html
Chopra, I., & Roberts, M. (2001). Tetracycline antibiotics: Mode of action, applications, molecular biology, and epidemiology of bacterial resistance. Microbiology and molecular biology reviews : MMBR. Retrieved February 2, 2023, from https://www.ncbi.nlm.nih.gov/pmc/articles/PMC99026/#:~:text=Tetracyclines%20are%20broad%2Dspectrum%20agents,and%20rickettsiae%2C%20and%20protozoan%20parasites
Ciancaglini, P., Simão, A. M. S., Bolean, M., Millán, J. L., Rigos, C. F., Yoneda, J. S., Colhone, M. C., & Stabeli, R. G. (2012). Proteoliposomes in nanobiotechnology. Biophysical Reviews, 4(1), 67–81. https://doi.org/10.1007/s12551-011-0065-4
Ekiert, D. C., Coudray, N., & Bhabha, G. (2022). Structure and mechanism of the bacterial lipid ABC transporter, MlaFEDB. Current Opinion in Structural Biology, 76, 102429. Exner, M., Bhattacharya, S., Christiansen, B., Gebel, J., Goroncy-Bermes, P., Hartemann, P., Heeg, P., Ilschner, C., Kramer, A., Larson, E., Merkens, W., Mielke, M., Oltmanns, P., Ross, B., Rotter, M., Schmithausen, R. M., Sonntag, H.-G., & Trautmann, M. (2017). Antibiotic resistance: What is so special about multidrug-resistant gram-negative bacteria? GMS hygiene and infection control. Retrieved February 2, 2023, from https://www.ncbi.nlm.nih.gov/pmc/articles/PMC5388835/
Falagas, M. E., Vouloumanou, E. K., Samonis, G., & Vardakas, K. Z. (2016). Fosfomycin. Clinical microbiology reviews. Retrieved February 2, 2023, from https://www.ncbi.nlm.nih.gov/pmc/articles/PMC4786888/#:~:text=Fosfomycin%20also%20exhibits%20considerable%20activity,(37%2C%E2%80%9341).
Hobot, J. A. (2015). Bacterial ultrastructure. Molecular Medical Microbiology, 7–32. https://doi.org/10.1016/b978-0-12-397169-2.00002-0
Hughes, G. W., Hall, S. C., Laxton, C. S., Sridhar, P., Mahadi, A. H., Hatton, C., … & Knowles, T. J. (2019). Evidence for phospholipid export from the bacterial inner membrane by the Mla ABC transport system. Nature microbiology, 4(10), 1692-1705.
Kamischke, C., Fan, J., Bergeron, J., Kulasekara, H. D., Dalebroux, Z. D., Burrell, A., Kollman, J. M., & Miller, S. I. (2019). The acinetobacter baumannii MLA system and glycerophospholipid transport to the outer membrane. Microbiology and Infectious Disease, 8. https://doi.org/10.7554/elife.40171
Libretexts. (2022). Gram-negative outer membrane. Biology LibreTexts. Retrieved from https://bio.libretexts.org/Bookshelves/Microbiology/Microbiology_(Boundless)/04%3A_Cell_Structure_of_Bacteria_Archaea_and_Eukaryotes/4.04%3A_Cell_Walls_of_Prokaryotes/4.4B%3A_Gram-Negative_Outer_Membrane
Locher, K. P. (2009). Structure and mechanism of ATP-binding cassette transporters. Philosophical Transactions of the Royal Society B: Biological Sciences, 364(1514), 239-245.
Malinverni, J. C., & Silhavy, T. J. (2009). An ABC transport system that maintains lipid asymmetry in the gram-negative outer membrane. Proceedings of the National Academy of Sciences, 106(19), 8009–8014. https://doi.org/10.1073/pnas.0903229106
Mann, D., Fan, J., Somboon, K., Farrell, D. P., Muenks, A., Tzokov, S. B., … & Bergeron, J. R. (2021). Structure and lipid dynamics in the maintenance of lipid asymmetry inner membrane complex of A. baumannii. Communications biology, 4(1), 817.
Silhavy, T. J., Kahne, D., & Walker, S. (2010). The bacterial cell envelope. Cold Spring Harbor Perspectives in Biology, 2(5). https://doi.org/10.1101/cshperspect.a000414
Tang, X., Chang, S., Qiao, W., Luo, Q., Chen, Y., Jia, Z., Coleman, J., Zhang, K., Wang, T., Zhang, Z., Zhang, C., Zhu, X., Wei, X., Dong, C., Zhang, X., & Dong, H. (2020). Structural insights into outer membrane asymmetry maintenance in gram-negative bacteria by MlaFEDB. Nature Structural & Molecular Biology, 28(1), 81–91. https://doi.org/10.1038/s41594-020-00532-y
Walsh, C., & Wencewicz, T. (2020). Antibiotics: challenges, mechanisms, opportunities. John Wiley & Sons.