Table of Contents
Immune Response and Immunological Memory Following SARS-CoV-2 Infection
Origin and Transmission of SARS-CoV-2
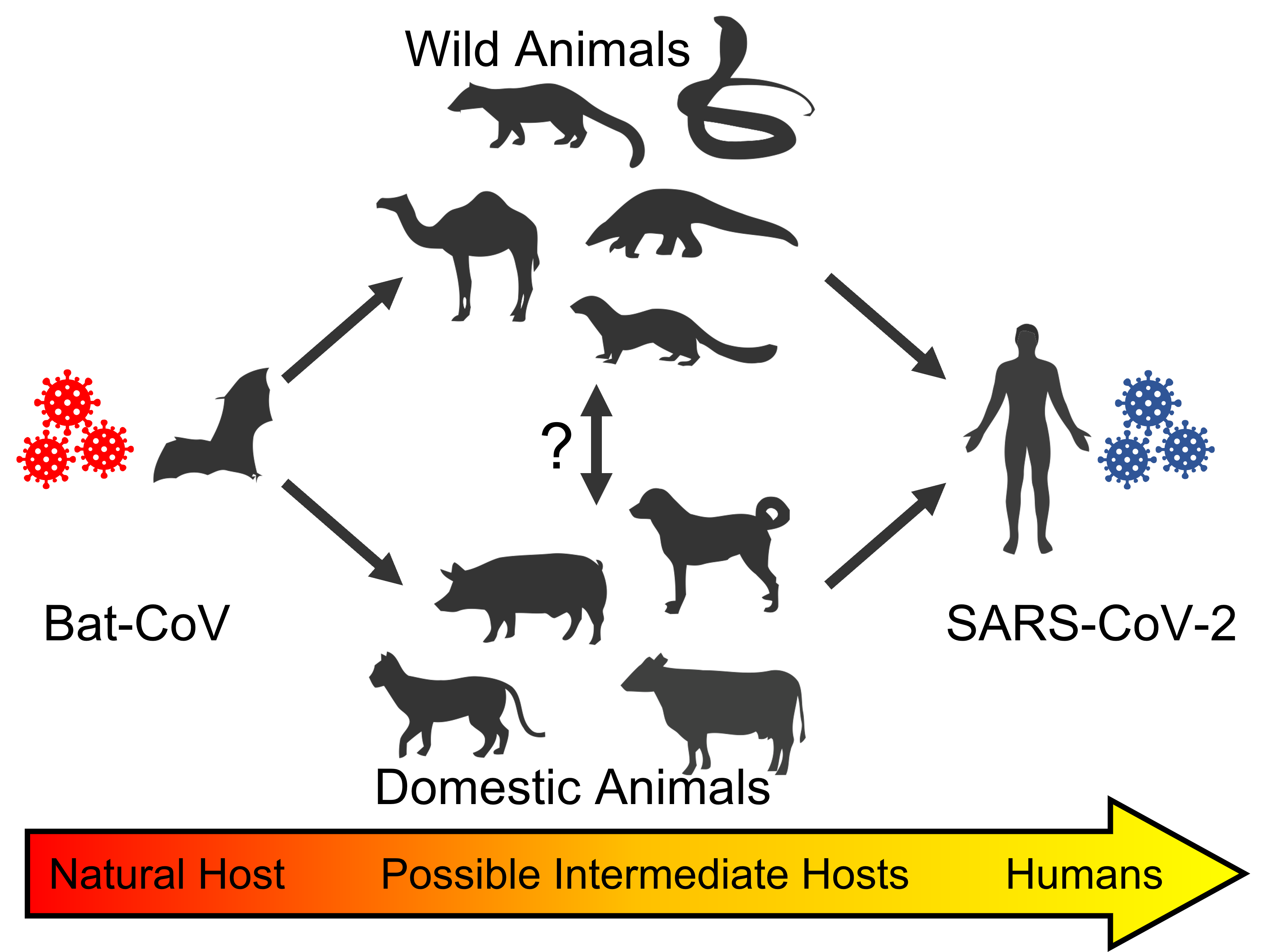
Figure 1: Potential transmission of SARS-CoV-2. Bats are the reservoirs of various coronaviruses (indicated as Bat-CoV). Bat-CoV-infected intermediate hosts are thought to have acquired mutations that permitted increased human transmission efficacy, culminating in zoonotic transmission to humans. These intermediate hosts are thought to include wild animals — such as camels, palm civets, pangolins, snakes, and minks — and domestic animals, like dogs, cats, pigs, and cows (Zhao, et al., 2020). Figure created using Biorender (2022).
Coronavirus disease 2019 (COVID-19), is a contagious disease that, since December 2019, is an ongoing pandemic (Asselah et al., 2020). COVID-19 traces its origins to Wuhan, China, where several health facilities reported pneumonia cases of unknown origin, with patients exhibiting symptoms including fever, dry cough, sore throat, difficulty breathing, muscle pain and fatigue (Asselah et al., 2020). Groups from China soon sequenced the causative virus, determining that it was a novel member of the betacoronavirus genus (Pedersen and Ho, 2020). The virus was later named severe acute respiratory syndrome-coronavirus-2 (SARS-CoV-2) by the International Committee on Taxonomy of Viruses (Rastogi et al, 2020).
Soon after its initial discovery, SARS-CoV-2 quickly spread worldwide, and as of January 22, 2022, there have been more than 362 million confirmed cases and 5.63 million deaths attributed to the disease ((Johns Hopkins University, 2022).
Before SARS-CoV-2, other coronavirus outbreaks in humans had also been documented, such as SARS-CoV in 2002-2003 and Middle East respiratory syndrome (MERS-CoV) in 2012, which were associated with case fatality rates of 9.6% and 34.4%, respectively (Abdelrahman, et al., 2020). Compared to these predecessors, however, SARS-CoV-2 exhibited a faster rate of human-to-human transmission, leading the World Health Organization to quickly declare its spread a worldwide public health emergency (Gao et al., 2020).
The closest known genetic relative of SARS-CoV-2 is bat coronavirus RaTG13, sharing 96.2% sequence identity (Wrobel et al., 2020; Abdelrahman, et al., 2020). This strong similarity suggests a zoonotic origin to the virus, whereby it was transmitted – indirectly or otherwise – from animals to humans. Although bats seem to be the reservoir host from which the virus originated, it is possible that other mammals serve as intermediate hosts, allowing SARS-CoV-2 to acquire the necessary mutations for effective human transmission. This is summarized in Figure 1 (Abdelrahman, et al., 2020).
Owing to the similarity in gene sequence among isolated patient SARS-CoV-2 samples, it is believed that the outbreak begun from a single individual point of origin, later spreading through person-to-person transmission. (Wang, et al., 2020; Chan et al., 2020). Early findings linked a number of COVID-19 patients to the Huanan Seafood Wholesale Market in Wuhan, which sells seafood and various exotic animal species (Shah, et al., 2020). Exotic animals, commonly sold in the market, have eating habits and habitats that render them likely carriers of various viruses and bacteria, possibly implicating the market in the virus’ zoonotic transmission (Shah, et al., 2020).
Human coronaviruses are respiratory viruses and are thus transmitted primarily through exhaled virus-containing respiratory droplets and aerosols (Harrison et al., 2020). For SARS-CoV-2 specifically, various other modes of transmission have also been proposed, including surface contamination and orofecal transmission (Harrison et al., 2020). However, a large proportion of the virus’ spread is thought to occur through airborne aerosol transmission by asymptomatic individuals, making the identification of infected individuals a challenge, as one can remain contagious for several days without showing any symptoms (Harrison et al., 2020). The virus primarily targets the lungs, entering through the respiratory tract, airway, and alveolar epithelium and quickly targeting associated cells for infection (Harrison et al., 2020).
Structure of SARS CoV-2
Figure 2: Structure and conformations of SARS-CoV-2. A. The SARS-CoV-2 virus (top) and the spike protein (bottom). The virus' membrane, envelope, spike, and genome-associated nucleocapsid proteins are shown. The spike is colour-coded according to the annotation below. The S1 subunit, which stretches from residues 1-685, includes the receptor-binding domain, while the S2 stretches from 686-1273. B. The up (receptor-accessible) and down (receptor-inaccessible) conformations of the S protein. Image adapted from: Huang, et al., 2020; Wrapp et al., 2020. Made using Biorender (2022).
SARS-CoV-2 is a single-stranded positive-sense RNA virus whose genome size is ~29.9 kb (Huang et al., 2020). The virus consists of four structural proteins, including the envelope protein (E), spike protein (S), membrane protein (M) and nucleocapsid protein (N), each encoded by their respective genes (Wang et al., 2020). The open reading frame (ORF) region encodes the remaining 16 non-structural proteins (often denoted as nsp1-16) (Wang, et al., 2020). Within the genome, the non-structural proteins comprise the first half, while the structural comprise the latter, as shown in Figure 3 (Yadav et al., 2021). As is typical of coronaviruses, SARS-CoV-2 assumes spherical shape and is enveloped with multiple spike glycoproteins projecting from the capsid, giving the appearance of a solar corona, from which the genus gets its name (Yadav et al., 2021). The four important structural proteins – S, E, M, and N – and their functions are described in further detail below:
Envelope protein (E): The envelope protein consists of five helical-shaped proteins bundled together to form a pentameric ion channel (Lalchhandama, 2020; Yadav et al., 2021). Each E protein has hydrophilic stretches along its N and C termini which flank a hydrophobic transmembrane domain (Yadav et al., 2021). While it is the smallest of the structural proteins, E plays a major role in pathogenicity, facilitating virion assembly, intracellular transport and budding (Lalchhandama, 2020; Yadav et al., 2021).
Membrane protein (M): Membrane proteins are the most abundant structural protein and, like the E proteins, are also involved in virion assembly and budding. (Schoeman & Fielding, 2019). The M proteins accomplish this by associating with other proteins, such as the nucleocapsid (Yadav et al., 2021). Membrane proteins possess three transmembrane domains are organized into homodimers (Yadav et al., 2021).
Nucleocapsid protein (N): Several nucleocapsid proteins form the nucleocapsid, which binds to the RNA genome in a beads-on-string conformation (Chang, et al., 2014). The protein consists of N- and C-terminal domains and an intermediate RNA-binding domain which interact to facilitate specific binding to viral RNA (Yadav et al., 2021). The N protein facilitates virion assembly and enhances viral transcription efficiency (Yadav et al., 2021).
Spike protein (S): The spike glycoproteins are crucial for entry into the host cells. S consists of two subunits, two subunits: S1 and S2, each comprising several functional domains (Walls et al., 2020). The S1 subunit includes the N-terminal domain, and a receptor-binding domain (RBD) (Yadav et al., 2021). The S2 contains the fusion peptide and several protein domains, including: the central helix, connecting, heptad repeat, and transmembrane domains, and a cytoplasmic tail. A furin cleavage site separates the S1 and S2 domain and is thought to undergo cleavage during the viral maturation process, triggering an irreversible conformational change in the spike which facilitates viral entry into host cells (Walls et al., 2020; Yadav et al., 2021; Jackson, 2022). SARS-CoV-2 and some domains within its spike protein are highlighted in Figure 2a.
In order to fulfill its function, S must take on a metastable state, where it is energetically stable yet also capable of conformationally transitioning into a lower-energy state (Jackson et al., 2022). This occurs as the receptor-binding domain of S1 undergoes hinge-like conformational changes that cause it to alternate between a receptor-inaccessible and accessible state, termed “down” and “up,” respectively (Wrapp et al., 2020). These conformations are contrasted in Figure 2b. This is necessary because the energy released during the conformational change is necessary to overcome the natural repulsion between the viral and cellular membranes, permitting close interaction of the RBD and its corresponding host receptor.
Mechanism of SARS-CoV-2 Viral Infection
Figure 3. Two distinct pathways of entry following ACE2 engagement. Left: Endosomal entry pathway where SARS-CoV-ACE2 complex is taken into the cell through endocytosis due to lack of interaction with TMPRSS2. The acidic environment of the endolysosome it is taken into leads to S2' cleavage-influenced viral RNA release into the cytoplasm. Right: Cell surface entry pathway where SARS-CoV-ACE2 complex interacts with TMPRSS2, leading to S2' cleavage-influenced viral RNA release into the cytoplasm. Image from Jackson et al., 2022.
Entry Process
SARS-CoV-2’s obligate cellular receptor, angiotensin converting enzyme (ACE2), is required for host cell recognition and binding (Shang et al., 2020). ACE2 – which is abundantly expressed on several surfaces, including the airway epithelia – catalyzes several physiologically useful reactions for the cardiovascular system and lungs. As it engages this receptor, the S protein’s subunits undergo conformational changes that bring the viral and cellular membranes together, creating a pore that links the viral genome to the cell cytoplasm (Jackson et al., 2022). This occurs as the S1 subunit engages with the ACE2, causing conformational changes that expose an internal cleavage site of the S2 subunit called S2’ (Shang et al., 2020). From here, viral entry can continue either through cell-surface or endosomal pathways:
Cell Surface Entry
Transmembrane protease, serine 2 (TMPRSS2) is a cell surface serine protease commonly co-opted by respiratory viruses to activate their glycoproteins. During cell surface entry, TMPRSS2 cleaves the S2’ site. (Jackson et al., 2022).
Endosomal Entry
If the cell of interest does not express enough of TMPRSS2, or if the SARS-CoV-2-ACE2 complex does not interact with a TMPRSS2, then the complex will instead be taken in through clathrin-mediated endocytosis (Jackson et al., 2022). This endocytosis is thought to be triggered by the presence of several SARS-CoV-2-bound ACE2 receptors (Jackson et al., 2022). Once endocytosed, lysosomes fuse with the endosome, forming endolysosomes which then undergo acidification (Jackson et al., 2022). This low pH environment activates cathepsins, namely cathepsin L, causing cleavage of the S2’ (Jackson et al., 2022).
Membrane Fusion and Viral Uncoating
Following S2’ cleavage, a fusion peptide is exposed and the dissociation of S1 from S2 occurs (Fehr & Perlman, 2015). This leads to a large conformational change for the S2 subunit that leads the fusion peptide into the target membrane (Jackson et al., 2022). This results in viral membrane fusion through the creation of a fusion pore (Jackson et al., 2022). SARS-CoV-2’s viral RNA is then allowed into the target cell’s cytoplasm in order to undergo uncoating as well as viral replication.

Figure 4. Annotated SARS-CoV-2 (+)ssRNA genome. From 5' to 3', non-structural proteins ORF1a and 1b are indicated, alongside structural proteins S, E, M, and N. Adapted from Yadav et al., 2021

Figure 5. Synthesis of complementary (-)ssRNA from the viral genome can either result in full genome synthesis or in the synthesis of nested mRNAs, the latter of which forms structural proteins that associate with the viral genome during assembly. Adapted from Flint et al., 2019.
Viral Gene Expression and RNA Synthesis
Genomic translation
After its release into the cytoplasm, viral genome expression is regulated both spatially and temporally (V’kovski et al., 2021). Open reading frame (ORF) 1a and open reading frame 1b from the genomic RNA encode polyproteins pp1a and pp1ab (V’kovski et al., 2021). Pp1a and pp1ab undergo autoproteolytic cleavage co- and post-translationally, releasing 16 different non-structural proteins (V’kovski et al., 2021). Pp1a releases nsp1-11, while pp1b releases nsp1-10/12-16 (V’kovski et al., 2021). Nsp1 inhibits translation within the host cell, both directly and indirectly by degrading host mRNAs (Thoms et al., 2020). Nsp2 through 16 create the replication and transcription complex (RTC) (V’kovski et al., 2019). Of these, nsp2 through nsp11 provide supporting functions for the viral RTC, such as: modulation of membranes in the cells, host immune evasion, and providing replication cofactors (V’kovski et al., 2019). Nsp12 through nsp16 underlie the key enzymatic functions in RNA synthesis, namely proofreading and modification (V’kovski et al. 2019). An annotation of the SARS-CoV-2 genome is shown in Figure 4.
Viral Genome Replication
A key element in SARS-CoV-2 replication is nsp12, which encodes the RNA-dependent RNA polymerase (RdRp) (Yadav et al., 2021). The RdRp is used to synthesize the complement of a giving RNA sequence, and in SARS-CoV-2, nsp12 achieves this function in association with two cofactors: nsp7 and nsp8 (Snider et al., 2016). Uniquely, coronaviruses have proofreading mechanisms, furnished in SARS-CoV-2 by nsp14, which also synthesizes the 5’ cap (Yadav al., 2021).
As a positive-sense single-stranded RNA virus, replication of SARS-CoV-2 involves the synthesis of full-length negative-sense genome copies using the RdRp. In coronaviruses and some others, this involves the production of several sub-genomic RNAs (sgRNAs); fragments of the original genome synthesized as a result for the RTC’s dissociation while copying the negative-sense strand (Flint et al., 2019). This results in a nested set of RNAs which share a common 5’ leader sequence and which encode other viral proteins, such as the structural proteins (Flint et al., 2019). To replicate its genome, the RTC synthesizes full-length negative-sense RNAs, which serve as the synthesis of full-length positive-sense strands (V’kovski et al., 2021).
Structural and Accessory Proteins
As it replicates, the membrane-bound proteins S, E, and M are inserted into the endoplasmic reticulum (Flint et al., 2019). N protein associates with the positive-sense mRNA, forming the viral genome (Flint et al., 2019). At the ER-Golgi intermediate compartment (ERGIC), the virus is assembled as the membrane-bound proteins associate with the genome (Flint et al., 2019). From there, most coronaviruses leave the infected cell through exocytosis, however, it is said that SARS-CoV-2 instead leaves infected through the lysosomal trafficking pathway (Ghosh et al., 2020). This process is summarized in Figure 5.
Innate Immunity
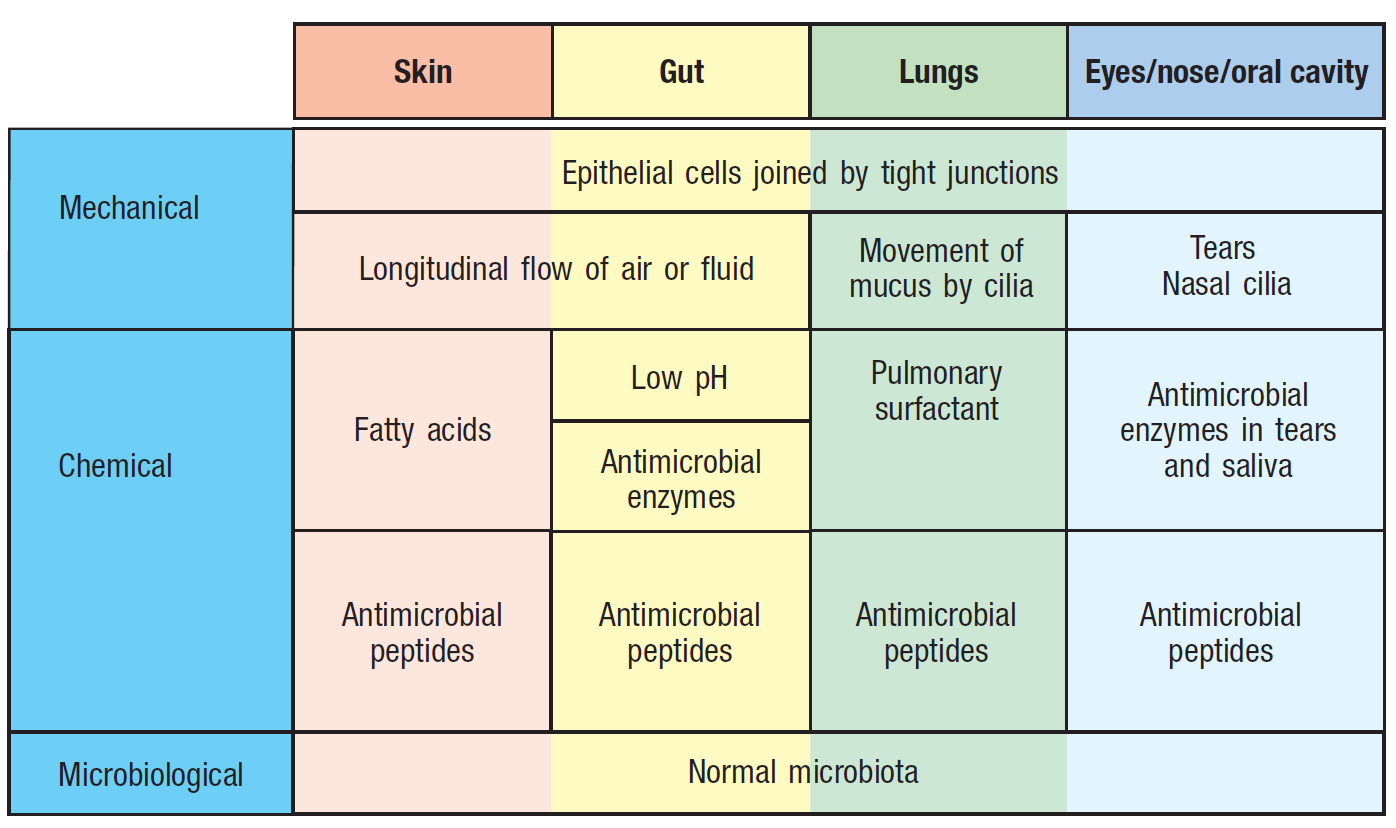
Figure 6. Epithelial barriers to infection include mechanical, chemical, and microbiological. These exist at all times and prevent the epithelium from colonization by pathogens. Image from Parham and Janeway, 2015.
Innate immunity is the body’s first line of defense in response to foreign antigens. Innate immunity includes immediate responses, which are ready at all times, and induced responses, which are mobilized upon detection of the infection. Immediate innate responses include:
- Physical barriers, such as the microbiota, mucus which is regularly turned over, tight junctions between epithelial cells, stomach acid, and antimicrobial peptides (Parham and Janeway, 2015). Collectively, these prevent the mucous membranes from colonization by bacteria, viruses and parasites (Parham and Janeway, 2015). These physical barriers to infection are further described in Figure 6.
- The complement system, which marks pathogens for destruction and recruits immune cells to the site of the pathogen (Parham and Janeway, 2015).
- Macrophages, which phagocytize invading micro-organisms, often working alongside the complement system (Parham and Janeway, 2015).
As its name implies, the induced response is activated in response to the infection (Parham and Janeway, 2015). Detection of the pathogen is facilitated through soluble and cellular pattern recognition receptors, which occur on immune cells and recognize shared structural features common to several pathogens (Parham and Janeway, 2015). Components of the induced response include cytokines, inflammation, and several immune cells (Parham and Janeway, 2015).
Cells of the Immune System
Immune cells are the backbone of both the innate and adaptive immune response. In the innate response, the cells involved include monocytes, macrophages, neutrophils and natural killer cells (Parham and Janeway, 2015).
- Monocytes are cells derived from the bone marrow which are able to further differentiate into macrophages and dendritic cells (Parham and Janeway, 2015). Their main function is to clear infections through phagocytosis – the uptake and breakdown of foreign material with digestive enzymes (Parham and Janeway, 2015). Monocytes and their progeny are typically found circulating in the blood and at areas where there is a higher likelihood of infection, such as the lining of the lungs (Parham and Janeway, 2015).
- Neutrophils, like macrophages, phagocytize micro-organisms (Parham and Janeway, 2015). Although smaller in size, they are more readily available and are usually the first cells to be recruited to an infected area. They are usually found in very high numbers circulating in the blood (Parham and Janeway, 2015).
- Natural killer cells detect infected cells, tumor cells and physiologically stressed cells through the NK cell synapse. Within the synapse, sufficient signaling from activating receptors and low signaling from inhibiting receptors commits the natural killer cell to destroy the target cell (Parham and Janeway, 2015). NK cells induce target cell death through apoptosis, secreting enzymes within granules that cause proteolytic attack on the target cell, causing DNA fragmentation and disrupting membrane integrity (Parham and Janeway, 2015). This programmed cell death minimizes the potential damage to the area and makes the healing and recovery process much faster (Parham and Janeway, 2015).
Cytokines
Cytokines are small soluble molecules used in communication among cells (Parham and Janeway, 2015). As these secreted molecules bind their receptors, they trigger signaling pathways that change the behaviour of the recipient cell. There are several types of cytokines, including:
- Pro-inflammatory cytokines, which create a state of inflammation in the infected tissue, causing changes – swelling, redness, pain, and heat – that permit immune cells to access these otherwise isolated tissues. For example, macrophages and dendritic cells can secrete cytokines like IL-1β and TNFα, which promote the inflammation response, illustrated in Figure 7 (Parham and Janeway, 2015).
- Immune effectors are directed to the site of infection due to chemokines, or chemoattractant cytokines. Cells with the receptor for a chemokine can move up its concentration gradient, ultimately arriving at the site of infection (Parham and Janeway, 2015).
- Interferons are a class of cytokines that are released upon viral infection. They are taken up by somatic and immune cells alike, instructing the cells to produce an anti-viral response, synthesizing anti-viral proteins and amplifying antigen presentation. There are 3 types of interferons that are secreted by cells, type I, type II and type III (Parham and Janeway, 2015). Type I is secreted by cells infected by the virus. Type II is mainly secreted by T cells and is responsible for promoting macrophage and natural killer cell activity (Parham and Janeway, 2015). Type III shares similar function with Type I interferon but has slower kinetics and tends to be less inflammatory (Parham and Janeway, 2015).
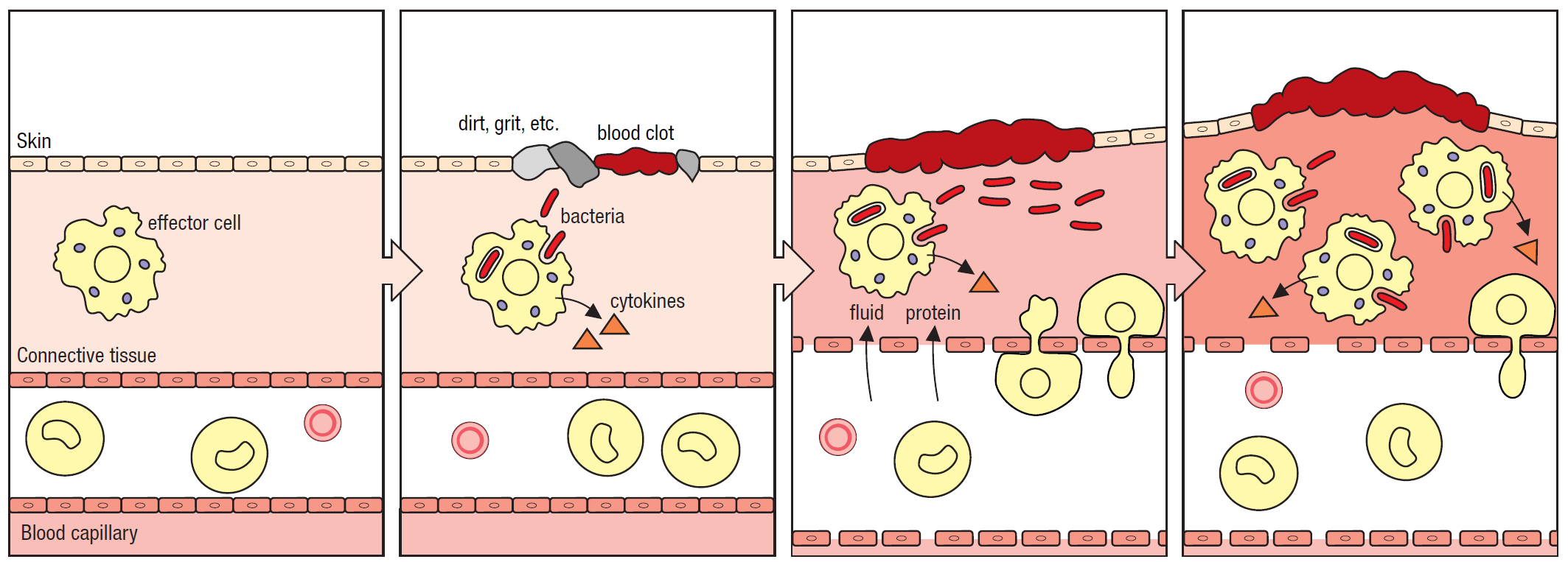
Figure 7. The inflammatory response. An inflammatory state is induced as pathogenic microbes are detected by effector cells resident within a tissue. In response, these cells secrete cytokines which induce the inflammatory state, increasing vascular permeability to permit inflammatory cells to access the tissue. Image from Parham and Janeway, 2015.
Adaptive Immunity
Overview
In cases where the innate immune system cannot clear the infection, it activates the second major arm of the immune system: adaptive immunity (Parham and Janeway, 2015). Unlike in innate immunity, where receptors are targeted to a broad range of pathogens, the adaptive immune response is focused on a specific pathogen, and the specificity of its targeting improves progressively throughout the infection (Parham and Janeway, 2015). The specificity and incremental improvement is achieved through several mechanisms which accelerate the rate of receptor evolution and select for cells expressing receptors that better target the antigen (Parham and Janeway, 2015).
Tissues of the Adaptive Immune System
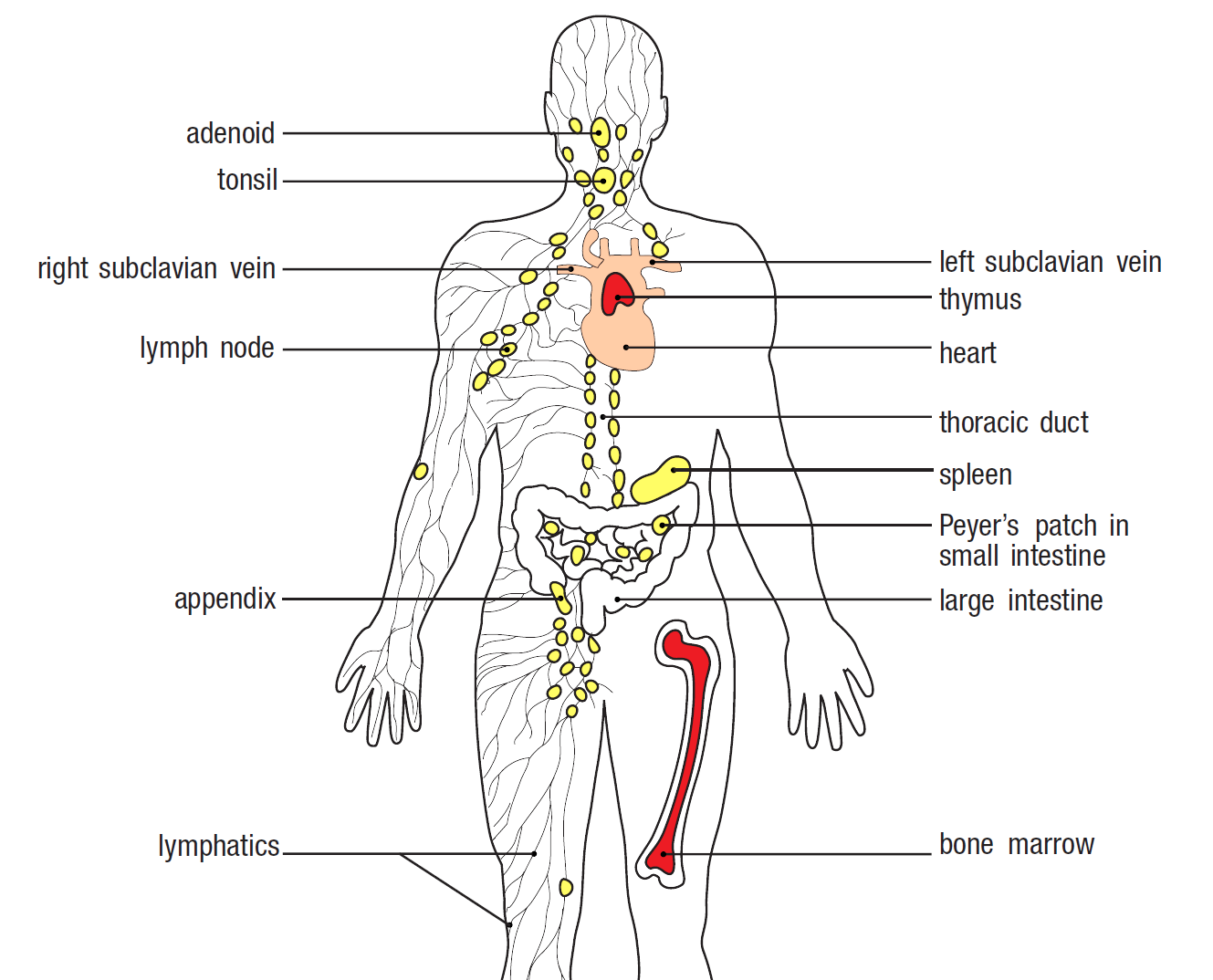
Figure 8. The body's lymphoid tissues. All lymphocytes are made in the bone marrow. B cells mature in the bone marrow, while T cells mature in the thymus. Primary lymphoid tissues are coloured red, secondary tissues are coloured yellow, and the branching lines represent the lymphatic vessels. Image from Parham and Janeway, 2015.
The adaptive immune system involves the lymphocytes, a type of white blood cell which includes several sub-lineages, most important of which are the T and B cells. These cells recognize features present on specific pathogens, called antigens, through their cell surface receptors, called immunoglobulins.
While lymphocytes circulate throughout the bloodstream, the vast majority occur within the lymphoid tissues and lymphoid organs. These tissues, alongside the circulating lymph fluid, comprise the lymphatic system. The major lymphoid organs include the bone marrow, thymus, spleen, adenoids, tonsils, appendix, lymph nodes, and Peyer’s patches. Primary lymphoid tissues are where the lymphocytes develop, and include the bone marrow and thymus. All other tissues are considered secondary lymphoid tissues and are where the naïve lymphocytes are converted into effectors. Effectors are distinguished from their naïve counterparts based on activation-induced markers; cell surface markers expressed in response to transcriptional changes associated with the cell’s activation. This could involve the expression of new markers on the cell surface or the removal of previously existing markers.
The respiratory and gastrointestinal tracts, because they come into constant contact with diverse populations of microorganisms, are especially prone to infection. Thus, these mucosal surfaces are often associated with secondary lymphoid tissues, collectively called the mucosa-associated lymphatic tissue.
Within the lymphatic system, lymph nodes are linked to each other by a series of lymph vessels, which carry the lymph fluid. Projecting from the lymph nodes are vessels for lymph and for blood. Uniquely, naïve lymphocytes can use the lymph nodes to move through the body in both blood and lymph.
Activation of the Adaptive Immune System
The activation of the adaptive immune response involves several steps and cell types (Parham and Janeway, 2015). Dendritic cells, a type of antigen-presenting cell, are responsible for activating the T cells, which in turn activate the B cells into plasma cells, which secrete antibodies (Parham and Janeway, 2015). The effector cells and their secreted components are carried out of the lymph node and towards the blood, where they target the infected tissues (Parham and Janeway, 2015).
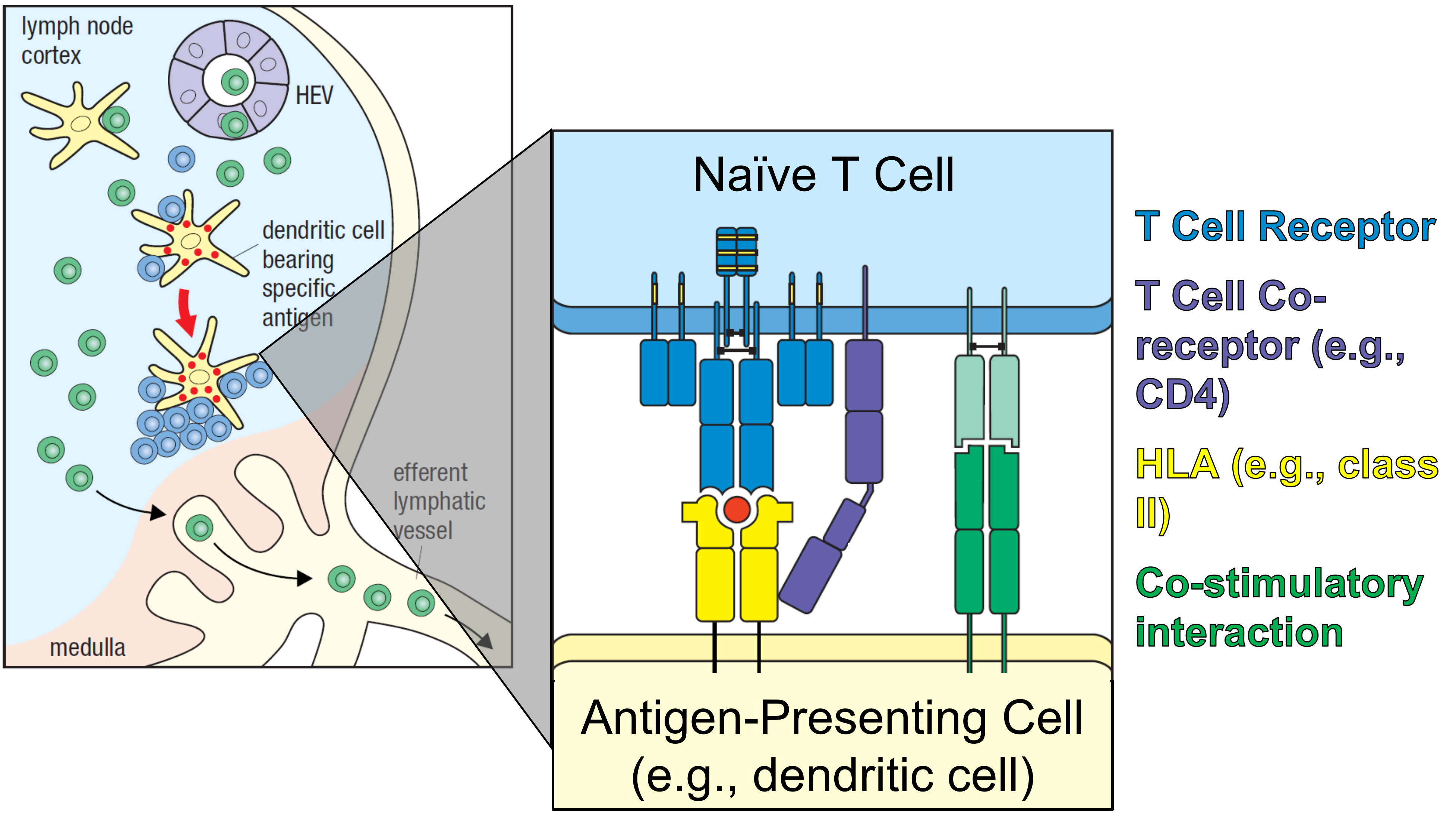
Figure 9. T cell activation. Within the T cell zone of the lymph node, naïve T cells are presented antigen on class II HLA by dendritic cells. Those with the cognate receptor to the HLA-antigen complex interact at the T cell synapse (shown on the right), using the T cell receptor, a co-receptor (either CD8 or CD4), and a co-stimulatory molecule. Together, these signals activate the naïve T cell into an effector form, either CD4+ or CD8+. Adapted from Parham and Janeway, 2015.
T Cell Activation
Naïve lymphocytes circulate throughout the bloodstream and may sometimes enter the lymph nodes, where they travel throughout the lymphatic system. As they enter lymphatic organs, the T and B cells segregate to different areas (Parham and Janeway, 2015). However, many other cells and cellular components can enter the lymphatic system, such as intact pathogens, their components, and antigen-presenting cells, such as dendritic cells (Parham and Janeway, 2015). As they sample the extracellular environment at the site of infection, or as they themselves become infected, dendritic cells come to present antigen at their surface (Parham and Janeway, 2015). This occurs by loading one of two cell surface receptors, called major histocompatibility complex proteins, or MHC. Human leukocyte antigen (HLA) is the MHC-encoded glycoprotein expressed on human cells (Mosaad, 2015). The primary role of the HLA system is to regulate the immune system (Mosaad, 2015).
Each HLA molecule can only bind a single antigen, and as this binding occurs, the charged receptor is brought to the cell surface, where it can interact with other cells (Parham and Janeway, 2015). HLA class I proteins can present antigens from intracellular pathogens – meaning that the cell itself has been infected – while class II presents proteolytically processed antigens derived from extracellular pathogens (Parham and Janeway, 2015). All nucleated cells can express antigen on HLA class I, but only professional antigen presenting cells – a distinction which includes dendritic cells, macrophages, and B cells – can express on HLA class II (Parham and Janeway, 2015).
The dendritic cell’s HLA proteins are recognized by naïve T cells within the lymph node (Parham and Janeway, 2015). These cells express receptors called clusters of differentiation, or CD proteins, at their surface (Parham and Janeway, 2015). These CD molecules are called T-cell co-receptors, as they work alongside the T cell receptor to amplify the intracellular signal transmitted upon detection of antigen (Parham and Janeway, 2015). Cytotoxic T cells are characterized by the expression of CD8, while helper T cells express protein CD4. At this point, however, the T cells are not yet matured, so they express both (Parham and Janeway, 2015). T cells expressing CD4 recognize antigen presented on HLA-II, while T cells expressing CD8 recognize antigen on HLA-I (Parham and Janeway, 2015).
Interactions between the T cell and charged HLA molecules on the dendritic cell occur across a T cell synapse (Parham and Janeway, 2015). Within the synapse, the receptors and co-receptors come into contact, initiating an intracellular signaling pathway that leads to the division, proliferation, and differentiation, transforming the naïve T cells into effectors (Parham and Janeway, 2015). As they mature, the naïve cells lose the co-receptor that was not used in their activation, differentiating them into either CD4+ or CD8+ T cells (Parham and Janeway, 2015).
CD8+ T cells, or killer T cells, are crucial in defending against intracellular infections, destroying infected cells (Parham and Janeway, 2015). Due to their destructive nature, these cells sometimes cannot be activated solely by the dendritic cells, also requiring the assistance of helper T cells (Parham and Janeway, 2015). Once activated, the cytotoxic T cells employ various cytotoxins and cytokines to kill virus-infected cells (Parham and Janeway, 2015).
CD4+ T cells, or helper T cells, are not directly involved in clearance of an infection (Parham and Janeway, 2015). Rather, they assist other immune cells in pathogen clearance. This occurs as the T cells secrete cytokines into the local environment, or milieu, influencing the differentiation of nearby cells through paracrine signaling. Depending on the type of pathogen, a different immune response may be better suited to its clearance (Parham and Janeway, 2015). For this reason, the helper T cell population is heterogeneous, and includes Th1, Th17, Th2, Tfh (T follicular helper), and Treg (T regulatory) cells (Parham and Janeway, 2015). Naïve T cells are converted into these mature CD4+ cells according to the local cytokine environment, which in turn activates different transcription factors and causes the cells themselves to secrete different cytokines (Parham and Janeway, 2015). This interaction is summarized in Figure 9.
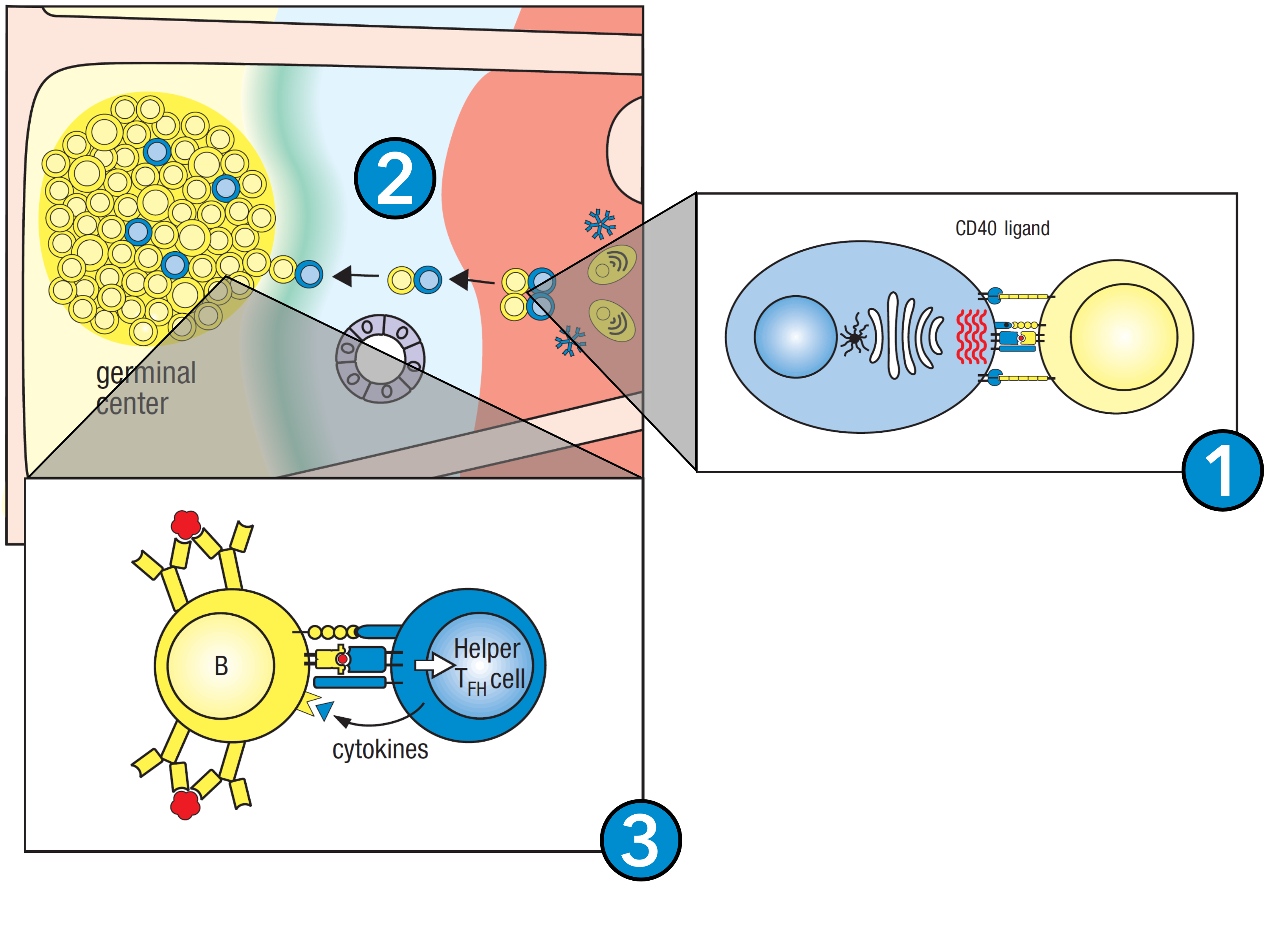
Figure 10. B cell activation. 1. Antigen-exposed B cells interact with nearby Tfh cells, activating them into effector B cells. 2. At this point, the B cells express only IgM and IgD, and thus travel towards the germinal center. 3. Within the germinal center, the B cells receive further signaling from Tfh cells and undergo isotype switching, giving rise to B cells expressing IgM, IgE, and/or IgA. Figure adapted from Parham and Janeway, 2015.
Cell-Mediated vs. Humoral Immunity
Depending on the type of infection, different cytokines are secreted in the local environment that drive the differentiation of the naïve T cells into effectors (Parham and Janeway, 2015). Based on the secreting cell, cytokines are sometimes divided into three classes (Parham and Janeway, 2015). When an immune response is strongly Th1-polarized, it is called cell-mediated immunity, as it involves pro-inflammatory cytokines and the recruitment of immune cells to the site of infection. The cytokines secreted by Th1 cells, which include IL-12 and IFN-gamma, are called type 1 cytokines (Parham and Janeway, 2015). Conversely, a Th2 polarized response involves extensive antibody secretion and thus contributes to humoral immunity, where the pathogen is neutralized indirectly (Parham and Janeway, 2015). The cytokines secreted by Th2 cells, IL-4 and IL-5, are considered type 2 cytokines (Parham and Janeway, 2015). Finally, type 3 cytokines include IL-17, IL-22, and IL-26, which mostly help to maintain homeostasis (Parham and Janeway, 2015).
B Cell Activation
Like T cells, B cells are activated as they bind their surface receptor to antigen within the lymph node (Parham and Janeway, 2015). B cells require three signals for activation. First, they require the cross-linking of the B-cell receptor by antigen (Parham and Janeway, 2015). Second, it requires the B-cell receptor to associate with the B-cell co-receptor, amplifying the B cell signal. Third and finally, almost all B cells can only be activated by binding with a CD4+ T follicular helper (Tfh) cell which is also specific for the antigen. As the B and T cells conjugate, the T cell secretes cytokines onto the B cell which cause its activation (Parham and Janeway, 2015). Once active, the B cell undergoes clonal expansion, producing a large population of expanding B cells within the lymph node, termed a germinal center (Parham and Janeway, 2015). Some of these germinal center B cells differentiate into plasma cells, which secrete their B cell receptors as soluble molecules called antibodies (Parham and Janeway, 2015). Others that are still bound to the Tfh cells undergo affinity maturation and isotype switching of their antibodies, allowing progressive refinement of the humoral response to infection (Parham and Janeway, 2015). This process is summarized in Figure 10.
Antibodies
Antibodies are the secreted form of the B-cell receptor / immunoglobulin (Parham and Janeway, 2015). They are produced by plasma cells, a type of effector B cell, in response to infection. Antibodies circulate in the blood and lymph and are frequently observed in the mucosa (Parham and Janeway, 2015). Antibodies provide strong protective immunity; the major goal of vaccination is to stimulate the production of neutralizing antibodies, which can directly inactivate a pathogen and prevent it from interacting with human cells. Each antibody molecule has specificity to only a single antigen or a small number of antigens, each potentially reflecting a different pathogen (Parham and Janeway, 2015). Antibodies are divided into a variable portion, which is specific to a particular antigen, and a constant portion, which interacts with other immune effectors (Parham and Janeway, 2015).
Based on their constant region, antibodies are grouped into five isotypes, or classes, each of which serves a different function (Parham and Janeway, 2015).
Antibody Isotype | Major Function(s) | Localization |
---|---|---|
Immunoglobulin M (IgM) | Activates the complement system | Blood and lymph |
Immunoglobulin D (IgD) | Sensitization of basophils (fights parasitic infections) | Co-expressed alongside IgM |
Immunoglobulin G (IgG) | Neutralization of pathogens, makes pathogens easier to phagocytize | Most abundant antibody in the internal body fluids, including blood and lymph |
Immunoglobulin A (IgA) | Neutralization of pathogens | Mucosa (e.g., gut lumen), breastmilk, saliva, sweat, tears |
Immunoglobulin E (IgE) | Sensitization of mast cells (produces histamine) | Skin, respiratory and gastrointestinal tracts |
The first immunoglobulins made by B cells are IgM and IgD, but once activated by the Tfh cells, the B cells undergo isotype switching, whereby they retain their variable region but switch the constant region, thus altering their specificity (Parham and Janeway, 2015).

Figure 11: An example flow cytometry dot plot readout. Naïve T cells, as they express both CD4 and CD8, would occupy the upper right quadrant. Cells expressing only one of the two would be either CD4+ or CD8+ T cells, and occupy the top left and bottom right quadrants, respectively. Figure adapted from Parham and Janeway, 2015.
Flow Cytometry and CD Factors
Cluster of differentiation markers are internationally established cell surface markers that are used to characterize cells by type (Parham and Janeway, 2015). There are many types of CD proteins, and they serve various purposes throughout the body (Parham and Janeway, 2015). The function and localization of a given CD marker can vary based on the organism in which it is identified (Parham and Janeway, 2015). Sometimes, a combination of CD markers are associated with a given phenotype (Parham and Janeway, 2015). Some example CD markers and their roles in humans include:
- Differentiation markers: CD69, CD103
- Growth factor receptor: CD25
- Macrophage activation: CD40
- Memory T cell marker: CD44, CD45RO, CD45RA, CCR7, CD127
- Resident cell marker: CD69, CD103
Cells expressing specific cell surface markers, such as CD markers, can be isolated from a bulk sample through flow cytometry (Parham and Janeway, 2015). Flow cytometry involves the use of reporter-conjugated antibodies to label cells expressing the markers of interest, then detecting the abundance of cells expressing or lacking the receptor of interest. Flow cytometry is usually used to distinguish a cell population based on the co-expression of two markers (Parham and Janeway, 2015). An example flow cytometry readout is shown in Figure 11.
Immunological Memory
Overview
Immunological memory is an important component of the adaptive immune system as it is necessary to respond quickly and effectively to pathogens that were previously encountered (Charles A Janeway et al., 2001). When an individual is initially exposed to a pathogen, the body initiates the primary immune response. After the primary immune response, antibodies, memory B cells, and plasma cells remain as the humoral components, while other immune effector cells are discarded (Gourley et al., 2004). Protective immunity and memory are now responsible for responding against the pathogen if it re-enters the body. This memory after infection or vaccination is centered around the creation of specialized memory cells that persist independently of the antigen remaining in the body (Farber et al., 2016). The memory immune cells must be specific for an antigen or epitope relating to the infecting pathogen (Farber et al., 2016). For immunological memory to be effective, the memory cells also need to be modified from the exposure to the antigen (Farber et al., 2016). These modifications can include faster reactivity to future encounters with the specific antigen or enhanced recruitment of other lymphocytes (Farber et al., 2016).
In addition to neutralizing antibodies, immunological memory also involves a cellular component, effected by CD4 and CD8 memory T cells (Gourley et al., 2004). Following antigen presentation to the T cell receptor and CD molecule, the memory cells exert the effector functions by either killing the infected cell directly or secreting cytokines to inhibit pathogen growth (Gourley et al., 2004). CD4 memory T cells are especially important in activating naïve B cells and helping CD8 T cells during the immune response (Gourley et al., 2004).
Vaccination, such as against SARS-CoV-2, relies on eliciting a memory response. Memory T and B cells created following antigen presentation allow for a rapid immune response upon SARS-CoV-2 infection. Immunity derived through vaccination is different from immunity following natural infection, as the circulating antibodies and memory cells are specific to multiple epitopes, rather than the single epitope used in vaccination.
Figure 13: Generation of memory B cells involves interaction with a follicular helper T cell in a germinal center reaction. In the germinal center, somatic hypermutation and affinity maturation occur which allow for the B cells to differentiate into memory B cells or long-lived plasma cells. Figure created using Biorender (2022).
Memory T cells
Antigen-specific B and T memory cell lineages constitute the immune memory system. The T memory cell lineage can be further broken down into tissue-resident, central, and effector memory cells (Gray et al., 2018). A memory T cell is generated from pathogen exposure to a naïve T cell which creates a large population of effector T cells. Differentiated effector T cells are short-lived and their purpose is to neutralize and kill the pathogen or virus. Once the pathogen or virus is cleared, most of the effector T cells go through apoptosis while a small subset remains as antigen-specific memory T cells. When exposed to the antigen of a past infection, the long-lived memory T cells can be quickly proliferated into more effector T cells to clear the infection. Tissue-resident memory (Trm) T cells are located in the peripheral tissues of the body and provide immediate protection upon pathogen entry (Gray et al., 2018). These cells are defined by the CD69 surface marker (Gray et al., 2018). Trm cells possess the knowledge of the initial infection site so they remain at this location, making them a more effective clearance method than the naïve T cells which patrol the lymphoid organs (Gray et al., 2018). Trm cells are further differentiated into CD4 and CD8 Trm cells. CD4 and CD8 Trm cells differ in their motility as CD8 cells are tethered to the epithelial cells through the CD103 integrin while the CD4 cells are more mobile (Gray et al., 2018). During respiratory infections, the presence of Trm cells in the lungs and lung-associated lymph nodes can provide localized protection (M.L. et al., 2021).
The central and effector memory T cells are found in circulation (Gray et al., 2018). Central memory (Tcm) T cells which express CCR7 and CD62L are primarily restricted to the secondary lymphoid tissues and the blood (Mueller et al., 2013). The effector memory T cells (Tem) lack CCR7 and CD62L, travel through the blood, and have the ability to cross into peripheral tissues (Gray et al., 2018). Unlike the tissue resident memory T cells, the effector memory T cells provide a slightly delayed response by migrating to the inflammatory area from various points in circulation to clear the pathogen/virus. Tcm cells produce interleukin-2 (IL-2) and proliferate, while Tem cells produce interferon-γ (IFN- γ) and are less proliferative (Mueller et al., 2013). These differences allow the Tcm cells to quickly produce antigen-specific CD4 T cells which can help B cells or migrate to the infection site (Gray et al., 2018). The Tcm cells do not have a direct function in killing the invader, but are involved in sustaining the overall immune response and supplying effector cells. The types of T cells are contrasted in Figure 12.
The activation of CD4 T cells is crucial to the adaptive response during infection. First to respond to are the Trm cells since they are located at the tissues being targeted. When a virus is re-encountered, clusters of CD4 Trm cells with macrophages and dendritic cells respond quickly by proliferating and releasing inflammatory cytokines and chemokines (Gray et al., 2018). Circulating CD4 Tem cells are then recruited to the inflammation site to issue the immune response (Gray et al., 2018). This leads to the activation of effector memory CD8 T cells which can directly kill cells infected with the virus. Then, the CD4 Tcm cells are activated in secondary lymphoid organs when dendritic cells or other APCs present them with the specific virus antigen (Mueller et al., 2013). The CD4 Tcm cells are responsible for amplifying the response systemically (Gray et al., 2018).
Memory B cells
Memory B cells are the other set of long-lived cells which can rapidly proliferate and generate bursts of antibody-secreting plasma cells when exposed to a recurring antigen. Memory B cells are formed when naïve B cells encounter an antigen and travel to the follicle of the secondary lymphoid organ to proliferate (Kurosaki et al., 2015). After proliferation, the activated B cells either differentiate into short-lived plasma cells in the marginal zone, or interact with follicular helper T cells which help to promote B cell receptor diversification through somatic hypermutation within a germinal center reaction (Weisel & Shlomchik, 2017). The B cells are then affinity-matured and can exit the germinal center as memory B cells or long-lived plasma cells (Kurosaki et al., 2015). These processes ensure memory B cells have high affinity for the specific antigen and can respond quickly in the context of the pathogen (Weisel & Shlomchik, 2017). This gives memory B cells a protective advantage over naïve B cells which have not undergone clonal expansion, differentiation, or affinity maturation, and therefore cannot divide quickly or produce high affinity antibodies (Weisel & Shlomchik, 2017). Antigen-specific memory B cells can be tissue-resident or circulate after T cell help and differentiation in the germinal center (M.L. et al., 2021). This process is summarized in Figure 13.
Conclusion
In summary, COVID-19 is an ongoing global pandemic caused by the positive-sense, single-stranded RNA virus SARS-CoV-2 (Flint et al., 2020). While the origin of the virus remains uncertain, it is thought to have involved a bat zoonosis, whereby the virus was transmitted to humans (Zhao et al., 2020). The virus co-opts the human ACE2 receptor for entry, then soon after uncoats and initiates its infectious cycle (Parham and Janeway, 2020). In response, the body leverages its innate and adaptive immune mechanisms to clear the infection. Once cleared, the origin, structure, and mechanism of infection together colour the memory response constructed by the adaptive immune system (Parham and Janeway, 2020). From there, the long-lived memory cells provide long-term, specific protection from subsequent exposures to the virus (Parham and Janeway, 2020).
Achieving a thorough understanding of the virus, its pathogenicity, and the immune response it elicits is necessary for expanding the body of knowledge surrounding SARS-CoV-2 and virology as a whole (Yadav et al., 2021). Translation of this knowledge, in turn, is essential in the development of new, evidence-based public health policies, as well as novel therapeutics and/or repurposing of existing drugs (Yadav et al., 2021). The fruits of past efforts were exemplified through the rapid development of various vaccine platforms, many of which have yielded promising results (Yadav et al., 2021).
References
Abdelrahman, Z., Li, M., & Wang, X. (2020). Comparative Review of SARS-CoV-2, SARS-CoV, MERS-CoV, and Influenza A Respiratory Viruses. Frontiers in Immunology, 11, 552909. https://doi.org/10.3389/fimmu.2020.552909
Asselah, T., Durantel, D., Pasmant, E., Lau, G., & Schinazi, R. F. (2021). COVID-19: Discovery, diagnostics and drug development. Journal of Hepatology, 74(1), 168–184. https://doi.org/10.1016/j.jhep.2020.09.031
Chang, C., Hou, M.-H., Chang, C.-F., Hsiao, C.-D., & Huang, T. (2014). The SARS coronavirus nucleocapsid protein – Forms and functions. Antiviral Research, 103, 39–50. https://doi.org/10.1016/j.antiviral.2013.12.009
COVID-19 Data Repository by the Center for Systems Science and Engineering (CSSE) at Johns Hopkins University. https://github.com/CSSEGISandData/COVID-19
Farber, D. L., Netea, M. G., Radbruch, A., Rajewsky, K., & Zinkernagel, R. M. (2016). Immunological memory: Lessons from the past and a look to the future. Nature Reviews Immunology, 16(2), 124–128. https://doi.org/10.1038/nri.2016.13
Fehr, A. R., & Perlman, S. (2015). Coronaviruses: An overview of their replication and pathogenesis. Methods in Molecular Biology (Clifton, N.J.), 1282, 1–23. https://doi.org/10.1007/978-1-4939-2438-7_1
Flint, J., Racaniello, V. R., Rall, G. F., Hatziioannou, T., & Skalka, A. M. (2020). Principles of Virology (5th edition). ASM Press.
Gao, Y., Yan, L., Huang, Y., Liu, F., Zhao, Y., Cao, L., Wang, T., Sun, Q., Ming, Z., Zhang, L., Ge, J., Zheng, L., Zhang, Y., Wang, H., Zhu, Y., Zhu, C., Hu, T., Hua, T., Zhang, B., … Rao, Z. (2020). Structure of the RNA-dependent RNA polymerase from COVID-19 virus. Science (New York, N.y.), 368(6492), 779–782. https://doi.org/10.1126/science.abb7498
Ghosh, S., Dellibovi-Ragheb, T., Pak, E., Qiu, Q., Fisher, M., Takvorian, P., Bleck, C., Hsu, V., Fehr, A., Perlman, S., Achar, S., Straus, M., Whittaker, G., de Haan, X., Altan-Bonnet, G., & Altan-Bonnet, N. (2020). β-Coronaviruses Use Lysosomal Organelles for Cellular Egress. SSRN Electronic Journal. https://doi.org/10.2139/ssrn.3654626
Gourley, T. S., Wherry, E. J., Masopust, D., & Ahmed, R. (2004). Generation and maintenance of immunological memory. Seminars in Immunology, 16(5), 323–333. https://doi.org/10.1016/j.smim.2004.08.013
Gray, J. I., Westerhof, L. M., & MacLeod, M. K. L. (2018). The roles of resident, central and effector memory CD4 T-cells in protective immunity following infection or vaccination. Immunology, 154(4), 574–581. https://doi.org/10.1111/imm.12929
Harrison, A. G., Lin, T., & Wang, P. (2020). Mechanisms of SARS-CoV-2 Transmission and Pathogenesis. Trends in Immunology, 41(12), 1100–1115. https://doi.org/10.1016/j.it.2020.10.004
Hoffmann, M., Kleine-Weber, H., & Pöhlmann, S. (2020). A Multibasic Cleavage Site in the Spike Protein of SARS-CoV-2 Is Essential for Infection of Human Lung Cells. Molecular Cell, 78(4), 779-784.e5. https://doi.org/10.1016/j.molcel.2020.04.022
Huang, Y., Yang, C., Xu, X., Xu, W., & Liu, S. (2020). Structural and functional properties of SARS-CoV-2 spike protein: Potential antivirus drug development for COVID-19. Acta Pharmacologica Sinica, 41(9), 1141–1149. https://doi.org/10.1038/s41401-020-0485-4
Jackson, C. B., Farzan, M., Chen, B., & Choe, H. (2022). Mechanisms of SARS-CoV-2 entry into cells. Nature Reviews Molecular Cell Biology, 23(1), 3–20. https://doi.org/10.1038/s41580-021-00418-x
Janeway, C. (Ed.). (2001). Immunobiology: The immune system in health and disease ; [animated CD-ROM inside] (5. ed). Garland Publ. [u.a.].
Kurosaki, T., Kometani, K., & Ise, W. (2015). Memory B cells. Nature Reviews Immunology, 15(3), 149–159. https://doi.org/10.1038/nri3802
Lalchhandama, K. (2020). The chronicles of coronaviruses: The electron microscope, the doughnut, and the spike. Science Vision, 20, 78–92. https://doi.org/10.33493/scivis.20.02.03
Lucey, D. R., Clerici, M., & Shearer, G. M. (1996). Type 1 and type 2 cytokine dysregulation in human infectious, neoplastic, and inflammatory diseases. Clinical Microbiology Reviews, 9(4), 532–562.
Mosaad, Y. M. (2015). Clinical Role of Human Leukocyte Antigen in Health and Disease. Scandinavian Journal of Immunology, 82(4), 283–306. https://doi.org/10.1111/sji.12329
Mueller, S. N., Gebhardt, T., Carbone, F. R., & Heath, W. R. (2013). Memory T Cell Subsets, Migration Patterns, and Tissue Residence. Annual Review of Immunology, 31(1), 137–161. https://doi.org/10.1146/annurev-immunol-032712-095954
Nakamura, T., Shirouzu, T., Nakata, K., Yoshimura, N., & Ushigome, H. (2019). The Role of Major Histocompatibility Complex in Organ Transplantation- Donor Specific Anti-Major Histocompatibility Complex Antibodies Analysis Goes to the Next Stage -. International Journal of Molecular Sciences, 20(18), 4544. https://doi.org/10.3390/ijms20184544
Parham, P., & Janeway, C. (2015). The immune system (Fourth edition). Garland Science, Taylor & Francis Group.
Pedersen, S. F., & Ho, Y.-C. (2020). SARS-CoV-2: A storm is raging. The Journal of Clinical Investigation, 130(5), 2202–2205. https://doi.org/10.1172/JCI137647
Poon, M. M. L., Rybkina, K., Kato, Y., Kubota, M., Matsumoto, R., Bloom, N. I., Zhang, Z., Hastie, K. M., Grifoni, A., Weiskopf, D., Wells, S. B., Ural, B. B., Lam, N., Szabo, P. A., Dogra, P., Lee, Y. S., Gray, J. I., Bradley, M. C., Brusko, M. A., … Farber, D. L. (2021). SARS-CoV-2 infection generates tissue-localized immunological memory in humans. Science Immunology, 6(65), eabl9105. https://doi.org/10.1126/sciimmunol.abl9105
Rastogi, M., Pandey, N., Shukla, A., & Singh, S. K. (2020). SARS coronavirus 2: From genome to infectome. Respiratory Research, 21(1), 318. https://doi.org/10.1186/s12931-020-01581-z
Schoeman, D., & Fielding, B. C. (2019). Coronavirus envelope protein: Current knowledge. Virology Journal, 16(1), 69. https://doi.org/10.1186/s12985-019-1182-0
Shah, V. K., Firmal, P., Alam, A., Ganguly, D., & Chattopadhyay, S. (2020). Overview of Immune Response During SARS-CoV-2 Infection: Lessons From the Past. Frontiers in Immunology, 11, 1949. https://doi.org/10.3389/fimmu.2020.01949
Shang, J., Ye, G., Shi, K., Wan, Y., Luo, C., Aihara, H., Geng, Q., Auerbach, A., & Li, F. (2020). Structural basis of receptor recognition by SARS-CoV-2. Nature, 581(7807), 221–224. https://doi.org/10.1038/s41586-020-2179-y
Snijder, E. J., Decroly, E., & Ziebuhr, J. (2016). The Nonstructural Proteins Directing Coronavirus RNA Synthesis and Processing. In Advances in Virus Research (Vol. 96, pp. 59–126). Elsevier. https://doi.org/10.1016/bs.aivir.2016.08.008
Thoms, M., Buschauer, R., Ameismeier, M., Koepke, L., Denk, T., Hirschenberger, M., Kratzat, H., Hayn, M., Mackens-Kiani, T., Cheng, J., Straub, J. H., Stürzel, C. M., Fröhlich, T., Berninghausen, O., Becker, T., Kirchhoff, F., Sparrer, K. M. J., & Beckmann, R. (2020). Structural basis for translational shutdown and immune evasion by the Nsp1 protein of SARS-CoV-2. Science, 369(6508), 1249–1255. https://doi.org/10.1126/science.abc8665
V’kovski, P., Kratzel, A., Steiner, S., Stalder, H., & Thiel, V. (2021). Coronavirus biology and replication: Implications for SARS-CoV-2. Nature Reviews Microbiology, 19(3), 155–170. https://doi.org/10.1038/s41579-020-00468-6
Walls, A. C., Park, Y.-J., Tortorici, M. A., Wall, A., McGuire, A. T., & Veesler, D. (2020). Structure, Function, and Antigenicity of the SARS-CoV-2 Spike Glycoprotein. Cell, 181(2), 281-292.e6. https://doi.org/10.1016/j.cell.2020.02.058
Wang, H., Li, X., Li, T., Zhang, S., Wang, L., Wu, X., & Liu, J. (2020). The genetic sequence, origin, and diagnosis of SARS-CoV-2. European Journal of Clinical Microbiology & Infectious Diseases, 39(9), 1629–1635. https://doi.org/10.1007/s10096-020-03899-4
Wang, M.-Y., Zhao, R., Gao, L.-J., Gao, X.-F., Wang, D.-P., & Cao, J.-M. (2020). SARS-CoV-2: Structure, Biology, and Structure-Based Therapeutics Development. Frontiers in Cellular and Infection Microbiology, 10, 587269. https://doi.org/10.3389/fcimb.2020.587269
Weisel, F., & Shlomchik, M. (2017). Memory B Cells of Mice and Humans. Annual Review of Immunology, 35(1), 255–284. https://doi.org/10.1146/annurev-immunol-041015-055531
Wrapp, D., Wang, N., Corbett, K. S., Goldsmith, J. A., Hsieh, C.-L., Abiona, O., Graham, B. S., & McLellan, J. S. (2020). Cryo-EM structure of the 2019-nCoV spike in the prefusion conformation. Science (New York, N.y.), 367(6483), 1260–1263. https://doi.org/10.1126/science.abb2507
Wrobel, A. G., Benton, D. J., Xu, P., Roustan, C., Martin, S. R., Rosenthal, P. B., Skehel, J. J., & Gamblin, S. J. (2020). SARS-CoV-2 and bat RaTG13 spike glycoprotein structures inform on virus evolution and furin-cleavage effects. Nature Structural & Molecular Biology, 27(8), 763–767. https://doi.org/10.1038/s41594-020-0468-7
Yadav, R., Chaudhary, J. K., Jain, N., Chaudhary, P. K., Khanra, S., Dhamija, P., Sharma, A., Kumar, A., & Handu, S. (2021). Role of Structural and Non-Structural Proteins and Therapeutic Targets of SARS-CoV-2 for COVID-19. Cells, 10(4), 821. https://doi.org/10.3390/cells10040821
Zhao, J., Cui, W., & Tian, B. (2020). The Potential Intermediate Hosts for SARS-CoV-2. Frontiers in Microbiology, 11, 580137. https://doi.org/10.3389/fmicb.2020.580137